10. Conserving Ecosystems
© 2019 J.W. Wilson and R.B. Primack, CC BY 4.0 https://doi.org/10.11647/OBP.0177.10
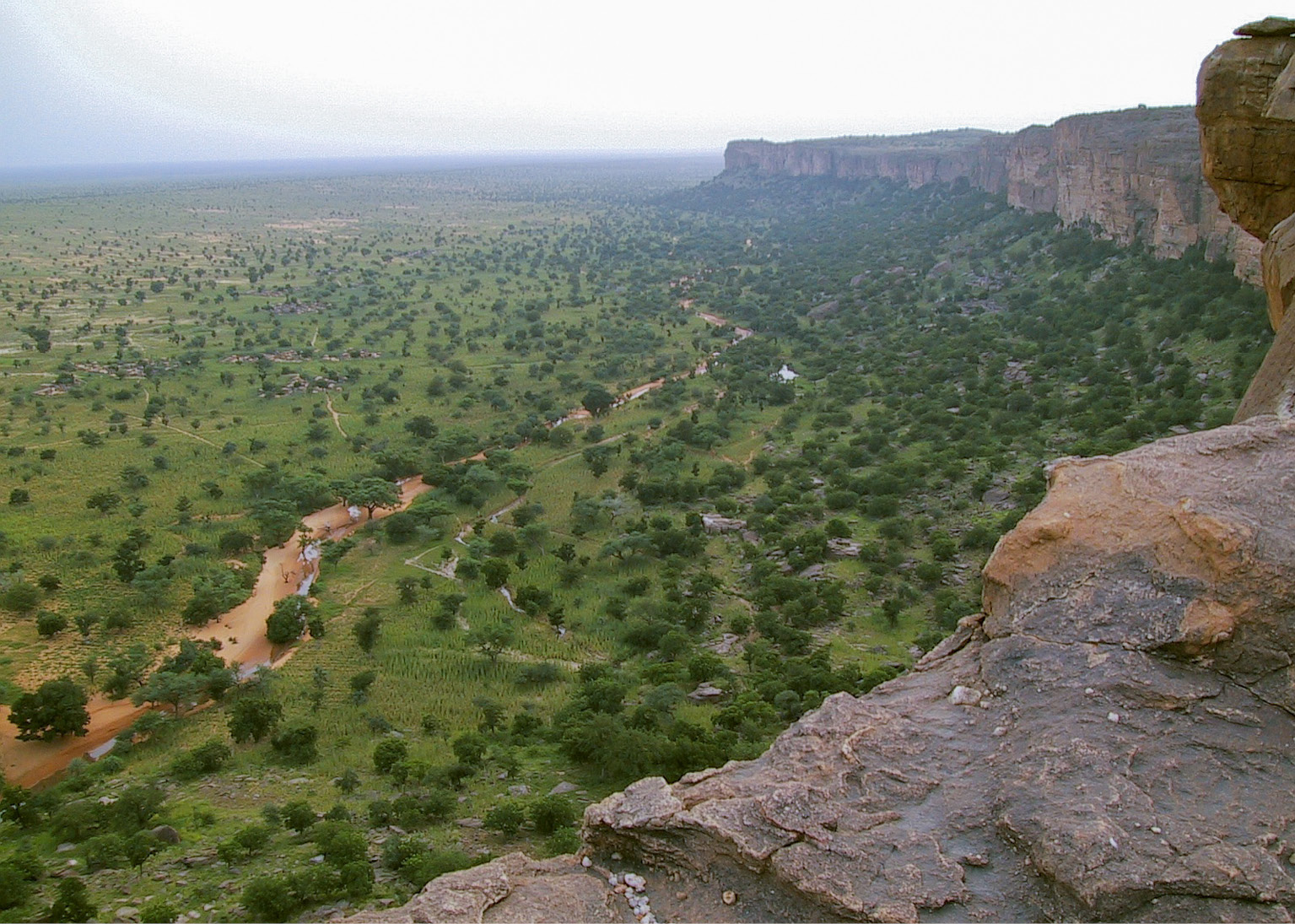
Rising 500 m above the Sahelian plains of Mali, the sandstone cliffs of the Bandiagara Escarpment are home to the Dogon people, whose unique homes are carved into the cliff’s walls. The Escarpment is a World Heritage Site, known for its outstanding cultural and natural value. However, both nature and humans are suffering from environmental degradation due to climate change and unsustainable land use. Photograph by Timm Guenther, https://commons.wikimedia.org/wiki/File:Les_Falaises_de_Bandiagara.jpg, CC BY-SA 3.0.
Habitat loss (and its associated degradation) is currently the most important threat facing Africa’s wildlife (Figure 10.1). When an ecosystem is destroyed or degraded, its ability to sustain wildlife is compromised, and the individuals that depend on that ecosystem for survival either need to adapt or move elsewhere, or they will die. Conversely, preventing ecosystem degradation and destruction is one of the single most important actions we can take to protect biodiversity. In the process, we also improve our own well-being, given that natural ecosystems are our first line of defence against natural disasters, and provide us with food, clean water, and other ecosystem services.
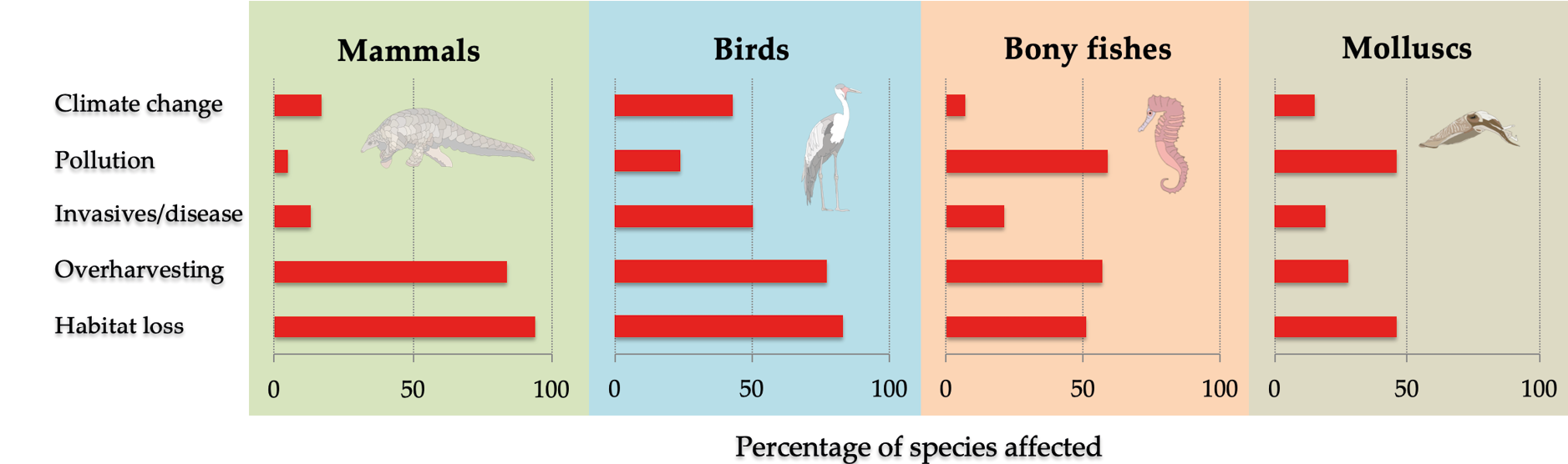
Figure 10.1 Habitat loss and degradation, much of which are driven by agriculture, are the most important threats to Africa’s wildlife, followed by overharvesting, invasive species and disease, and pollution. Groups of species often face similar threats: mammals and birds are more likely to be threatened by habitat loss, while fish and molluscs are more likely to be threatened by pollution. Percentages add up to more than 100% because many species face multiple threats. The influence of climate change is under-estimated because its impact on most species still need to be assessed. Source: IUCN, 2019, CC BY 4.0.
Broadly speaking, ecosystem conservation involves three different activities: (1) monitoring ecosystems, (2) maintaining ecosystems, and (3) restoring damaged ecosystems. While many books have been written on each of these three activities, the broad overview this chapter provides will hopefully enable readers to gain a basic understanding of the tools and methods used in ecosystem conservation.
10.1 Ecosystem Monitoring
A complex and adaptive ecosystem in which all the chemical, physical, and biological components, functions, and processes are intact and functioning normally is considered a healthy ecosystem (but see Cumming and Cumming (2015), for a discussion on this value-based term). In contrast, disturbing any of an ecosystem’s components, functions, and/or processes will, by definition, alter them to some degree (Table 10.1). In many cases, ecosystems that have been exposed to certain forms and levels of disturbances remain healthy because there is redundancy in the roles performed by different ecosystem components (Section 4.2.1). This ability of an ecosystem to withstand certain forms and levels of disturbances is referred to as ecosystem stability. Ecosystem stability could be the result of one or both of two qualities: resistance and resilience. Resistance is the ability of an ecosystem to retain the same characteristic communities and natural cycles throughout and after a disturbance event, while resilience is the ability of an ecosystem to rapidly recover or adapt after a disturbance event. For example, if the number of native aquatic insect species decline after non-native fishes are introduced to previously fish-free ponds, the pond’s ecosystem has low resistance. But if the native insect community recovers rapidly after the non-native fishes were removed, the ecosystem is resilient.
Table 10.1 Three ways how humans have changed the natural world.
Natural function |
Changes attributed to human activities |
Land surface |
As much as half of the world’s ice-free land surface has been transformed to cater to people’s need for natural resources. Much of these changes are driven by agricultural activities. |
Nitrogen cycle |
Human activities release massive amounts of nitrogen into natural ecosystems on a daily basis. Much of this occurs through the use of nitrogen fertilisers, burning fossil fuels, and cultivating nitrogen-fixing crops. |
Atmospheric carbon cycle |
Scientists estimate that humans would have doubled levels of carbon dioxide in the Earth’s atmosphere by the middle of this century. This is primarily the result of fossil fuel use and deforestation. |
Wildlife populations |
Between 1970 and 2014, Sub-Saharan Africa have lost three quarters of its freshwater vertebrates; the rate of these declines shows no sign of reducing. |
Pollutants |
Pollution from human activities have become so omnipresent that it is hard to escape its impacts. Microplastics have been found in drinking water and the food we eat (Chapter 7). |
Sources: MEA, 2005; Kulkarni et al., 2008; http://www.livingplanetindex.org
Many forms of ecosystem disturbances are easy to observe. Consequently, monitoring these visible forms of disturbances—such as the outright destruction of a forest or plastic pollution on a beach—focuses less on detection and more on developing systematic survey protocols (Section 9.1) that can provide information on whether a disturbance is spreading and increasing in intensity, or whether conservation action is successful in containing the threat. However, some disturbances are subtler, unobtrusive, and thus difficult to detect; examples include pesticide drift and agricultural runoff (Section 7.1). Adopting a “wait and see” approach to detecting these invisible forms of disturbances can be particularly damaging, since that approach generally ends at a point where the harm will either be impossible to reverse or will require significantly more resources and time than would have been the case if the problem was addressed earlier. In this way, there are many similarities between monitoring ecosystem health and human health—some ailments are easier to diagnose than others, but we avoid the worst-case scenarios by screening regularly for diseases and treating the threatening ones promptly.
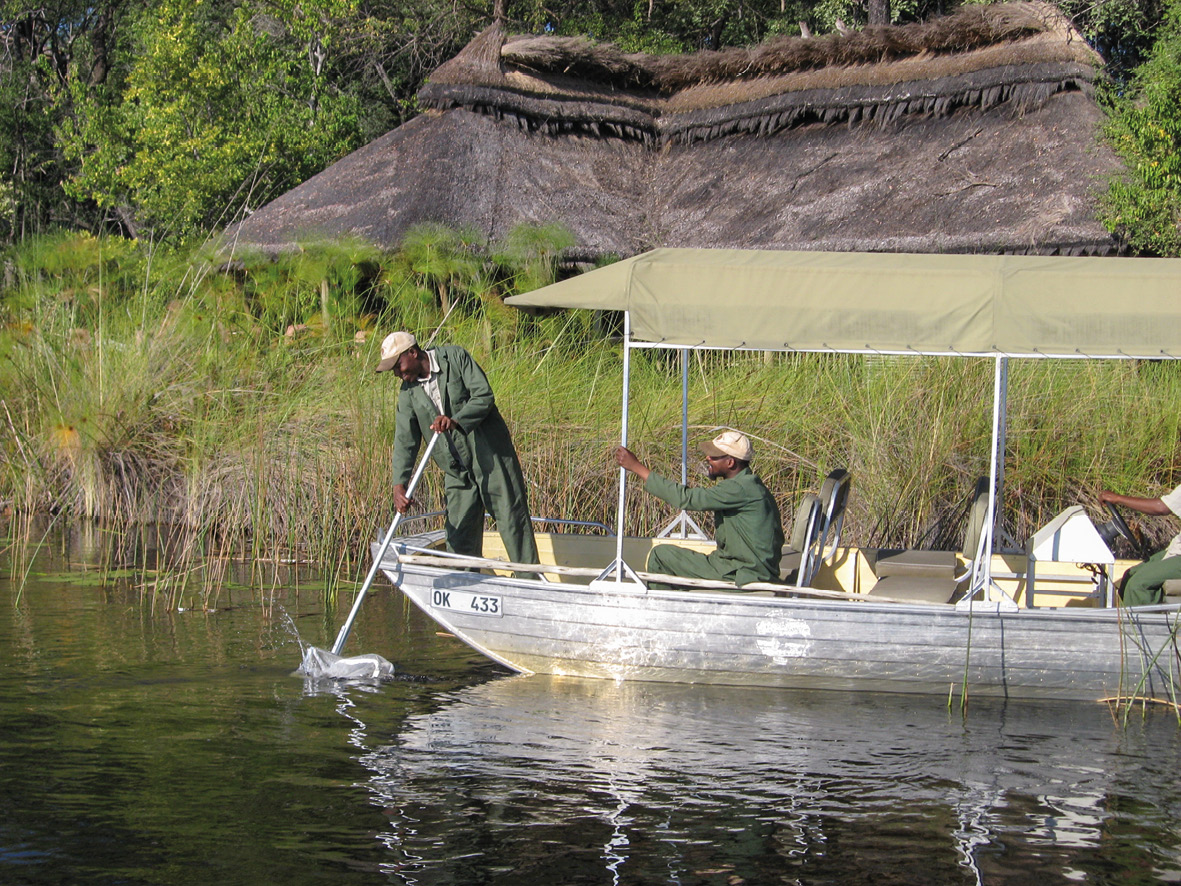
Figure 10.2 Biologists sampling aquatic macroinvertebrates in the Okavango Delta, Botswana, as part of a biomonitoring project of the Freshwater Research Centre. Photograph by Helen Dallas/FRCSA, CC BY 4.0.
Perhaps the most popular method conservation biologists use to monitor ecosystem health is known as biomonitoring. By monitoring the abundance and/or fitness of sensitive species (Box 10.1), biologists can sometimes detect ecosystem degradation before it becomes apparent to the human eye or escalates to a point where it starts impacting human lives (Bornman and Bouwman, 2012). Monitoring environmental indicators such as macroinvertebrates (Figure 10.2) is particularly popular when examining the ecological condition of aquatic ecosystems; mayflies, caddisflies, and stoneflies—specialists of undisturbed streams—are often replaced by flies and midges in polluted and disturbed environments. Sometimes however, when plants or animals are not easily monitored, certain aspects of those species can still be monitored. One example is monitoring total plant biomass as a proxy for soil nutrients or intensity of herbivory. Another option is to perform a bioassay, during which a sensitive organism (typically water fleas or plankton) is released into a potentially contaminated environment to see if death or declining health occurs.
By monitoring the abundance and/or fitness of sensitive species, biologists can detect threats to biodiversity before it becomes apparent to the human eye.
Box 10.1 Using Insects to Monitor Environmental Health
Department of Animal Biology and Conservation Science,
University of Ghana,
Legon, Ghana.
rkyerematen@ug.edu.gh
Insects are important to nearly every terrestrial food web in the world and serve a multitude of different purposes: some insects are responsible for pollination of plants while others are scavengers that clean up dead plant and animal material. In some cases, our understanding of an insect species’ ecological role can make it suitable as an indicator of environmental health. Biomonitoring looks at the presence and abundance of organisms within their natural communities to assess the impact of environmental disturbances; this knowledge can then be used to guide ecosystem management. An indicator taxon is one whose impact can be specifically and precisely measured; its abundance serves as a measure of the overall health of an ecosystem. Understanding how the presence and abundance of indicator species, and the relative abundance of tolerant and intolerant species, reflects the relative health of an environment can allow for rapid surveys of impaired ecosystems to assess trends as well as to track changes following remediation and restoration efforts.
Over the past few years, biomonitoring with insects as indicator taxa has become increasingly popular in Ghana. Butterflies are especially popular because they show varying relative sensitivities to environmental change; the abundance of certain butterfly species can for example be used to study the impact of habitat loss, fragmentation, and climate change (Kyerematen et al., 2018). The presence and abundance of butterflies more characteristic of open and disturbed ecosystems (Figure 10.A) can, for example, be used as an indicator of forest degradation.
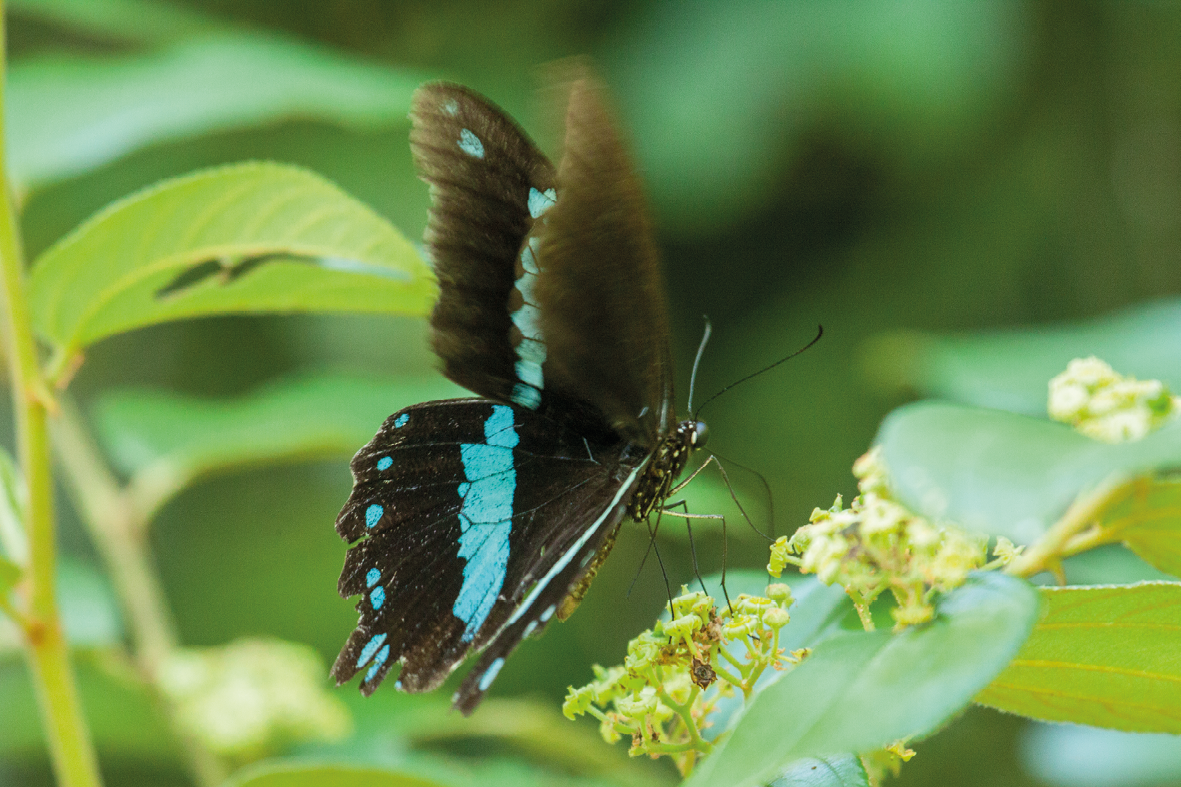
Figure 10.A Because the green-banded swallowtail (Papilio nireus) prefers open woodlands, researchers in Ghana are using their abundances in forests as a measure of habitat degradation. Photograph by Celesta von Chamier, CC BY 4.0.
Aquatic insects, particularly benthic macroinvertebrates, are also useful bioindicators. Freshwater resources, such as lakes and rivers, provide water for drinking and washing to local people, and a home for economically important taxa, such as fish and shellfish. Protecting these water sources is therefore important for safeguarding people’s health and livelihoods. The presence, absence, and diversity of certain benthic macroinvertebrates, even at the order level, can provide valuable information about whether a waterbody is being degraded or not (Kyerematen et al., 2014; Nnoli et al., 2019). A recent study showed that dragonfly and damselfly diversities and populations along the coastal Densu River in Ghana vary widely depending on the physical condition of the river and surrounding area (Acquah-Lamptey et al., 2013).
With their high diversity and varying tolerances for ecosystem conditions, insects are extraordinarily suited as ecological indicators in environmental monitoring. Each insect species is also part of a wider biological community with important ecological roles. If lost, not only will an abundance of other life be affected, such a loss may also hint at a looming crisis facing people living in those compromised ecosystems.
At times, conservation biologists may need to measure the physical environment to assess environmental health. This approach is particularly common when tracking pollution, for example by monitoring for changes in biochemical indicators. For example, measuring total phosphorus, nitrogen, and dissolved oxygen load in streams and other surface water can help scientists track eutrophication (Section 7.1.1). Measuring these and other biochemical indicators is usually accomplished directly via chemical analysis of environmental samples, such as soil and water. Sometimes however, biochemicals indicators are tracked indirectly via biological samples obtained from plants and animals. Because they bio-accumulate heavy metals and other pollutants, filter feeders such as clams and mussels (e.g. Bodin et al., 2013) are particularly useful in this regard as they can be used to detect very low concentrations of harmful chemicals in the environment.
10.1.1 Monitoring ecosystems with geospatial analysis
A persistent challenge facing biologists who monitor ecosystems—and other aspects of biodiversity—is achieving consistency across space and time. Consider a survey of a sensitive bird community to track ecosystem change; not only will different observers have varying levels of experience, but they will almost certainly see different birds during an early morning census compared to one later in the afternoon due to differences in biology and behaviour between species. These factors introduce error into monitoring data, which in turn can mask the effect a biologist tries to measure (Buckland and Johnston, 2017). Laboratory scientists’ control for these confounding factors by making multiple measurements under strictly controlled conditions. But for conservation biologists working outside in the wind and rain, repeated observations under similar conditions can be near impossible.
Geospatial analysis offers a variety of tools that allow biologists to overcome some of these traditional field monitoring challenges. These tools use geographic information systems (GIS) computer software packages to store, display, and manipulate a wide variety of data representing the natural environment, biodiversity, and human land-use patterns as they relate to one another on Earth’s surface. GIS thereby allows biologists to easily visualise and analyse spatial relationships between mapped data, which may include aspects such as vegetation types, climate, soils, topography, geology, water availability, species distributions, existing protected areas, human settlements, and human resource use (Figure 10.3). Understanding such relationships helps conservation biologists to prioritise their actions, for example by identifying areas where data are lacking, where an environmental change requires further investigation, or where gaps in regional protected areas network exist.
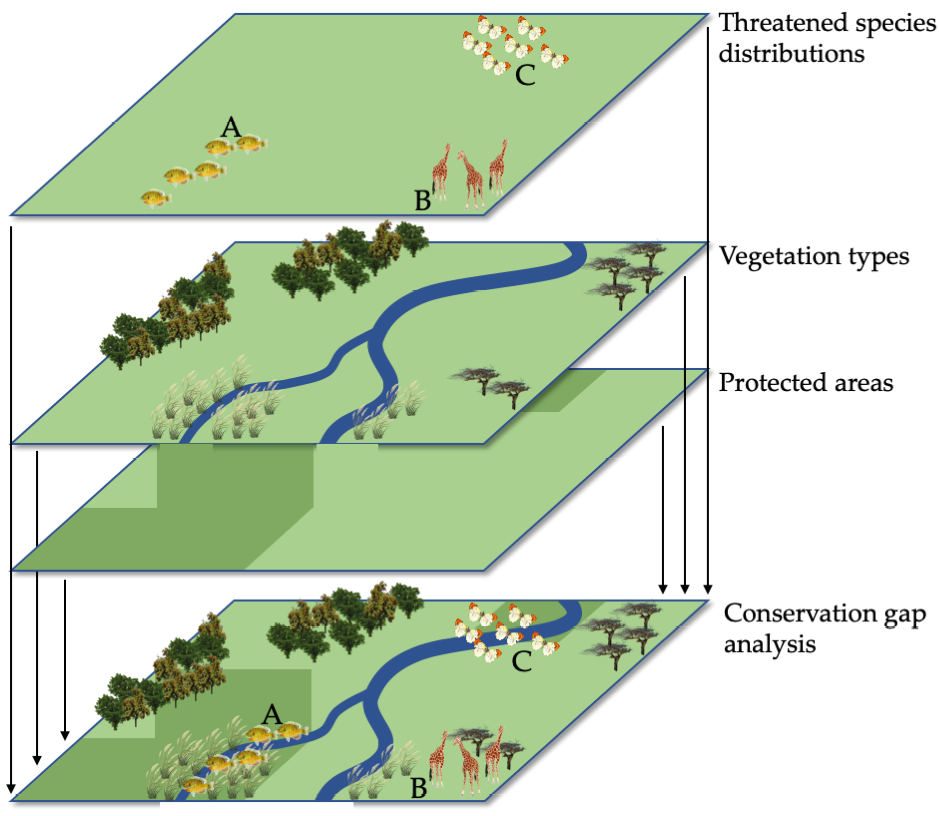
Figure 10.3 Distribution maps for three threatened species are overlaid onto maps of protected areas and vegetation types to identify potential conservation gaps (discussed in Chapter 13). This GIS analysis shows that Species A is well protected, Species B is protected to some extent, and Species C is not protected at all. Species C would thus be considered a high conservation priority. After Scott et al., 1991, CC BY 4.0.
Remote sensing is a special branch of geospatial analysis directed at obtaining ecosystem data without making physical contact (i.e. boots on the ground) with the observation site. Before the turn of the 20th century, the most popular form of remote sensing was aerial photography from airplanes. These aerial photographs facilitated geographers’ ability to draw maps of landscape features, including human infrastructure and natural vegetation patterns. Remote sensing opportunities greatly expanded from 1960 onward, with the launch of the National Aeronautical Space Administration’s (NASA) first Earth observation satellites, to take photographs of Earth from space for weather forecasting. Subsequent satellite programmes expanded their scope to also collect additional data of Earth’s surface and atmosphere. While much of this data would have been useful to conservation, early satellite data products were very expensive and thus largely out of reach of the larger conservation community. This all changed in 2008 when NASA started distributing their Earth observation products for free to the public, heralding an era in which remote sensing became a standard tool in the conservation field.
Remote sensing offers a variety of tools that allow biologists to monitor biodiversity beyond the abilities of traditional field monitoring techniques.
Today, hundreds of Earth observation satellites circle the planet, offering near real-time access to unbiased and consistent environmental datasets of nearly all terrestrial surfaces, oceanic surfaces and floor depths, and the atmosphere, all from the comfort of a computer connected to the internet (Wilson et al., 2013). Scientists use these products in ecosystem monitoring efforts, including monitoring water quality (Dube et al. 2015), forest loss (Laporte et al., 2007), coral reef health (McClanahan et al., 2011), desertification (Symeonakis et al., 2004), and fire regimes (Archbald et al., 2010). Linking the information obtained by Earth observation satellites to biological information collected on the ground has proved invaluable in monitoring species’ threat statuses (Di Marco et al., 2014), ecosystem connectivity (Wegmann et al., 2014), and habitat suitability (Torres et al., 2010), as well as understanding how biodiversity responds to environmental changes (Box 10.2).
Box 10.2 Remote Sensing and Spatial Analysis for African Conservation
Global Change Institute (GCI), University of the Witwatersrand,
Johannesburg, South Africa.
Barend.Erasmus@wits.ac.za
Remote sensing is the art and science of observing objects or landscapes from a distance, without being in direct contact with the environment. Although you can think of wildlife photography as a type of remote sensing, the term usually refers to aerial photography (Figure 10.B), or images taken via satellite. For both cases, there is a trade-off between the area covered by each photo (the footprint), how much digital storage space is available, and how often a satellite takes a picture of the same area. For satellites that take pictures of the earth systematically, along a pre-defined path, cloud cover determines how often you can get a usable image. Recent improvements in technology, now allow for the deployment of constellations of satellites that have a collective point-and-track capability to observe an area almost continuosly.
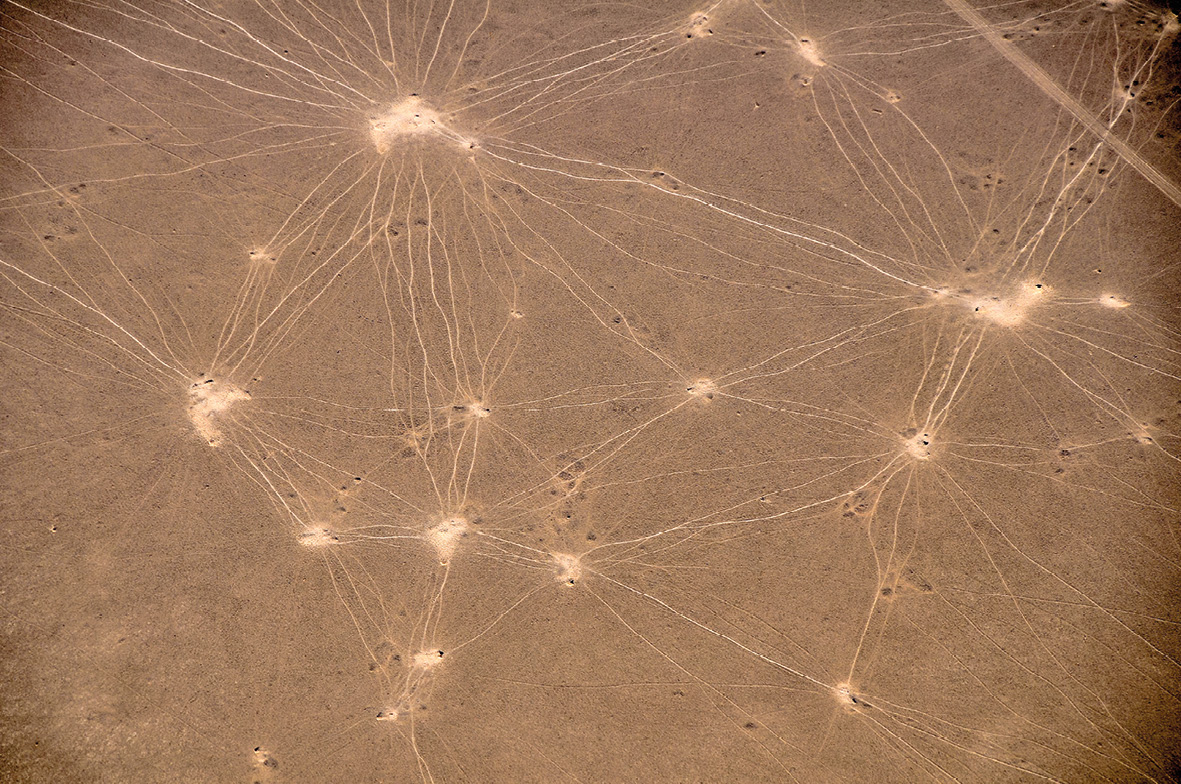
Figure 10.B Aerial photograph of Kaa pan in the southwestern Kalahari region of Botswana. Gemsbok (Oryx gazella, LC), red hartebeest (Alcelaphus buselaphus caama, LC), and eland (Tragelaphus oryx, LC) excavate holes for lekking (eating soil with high salt content), and the footpaths between the different lekking sites clearly show preferences for certain sites. In the same way, on a much broader scales, ungulates move across landscapes to access other resources, such as water and grass, whilst avoiding human hunters and predators. Photograph by B.F.N. Erasmus, CC BY 4.0.
Our eyes are sensitive to the colours red, green, and blue, together called the visible part of the electromagnetic spectrum. This is actually a very small part of the entire spectrum, and scientists have found, for example, that vegetation seen as uniformly green shows a lot of variation in the infrared part of the spectrum. For this reason, many satellites carry cameras that can “see” infrared light, and by proxy, measure vegetation health, biomass, and sometimes even structure. This capability, together with frequent revisit rates, allows for a unique view of how African landscapes change, whether through habitat loss, seasonal changes, or drought. Analyses of 13 years of remotely sensed data show that the most arid parts of southwest Botswana now experience typical summer vegetation conditions later in the year (Dubovyk et al., 2015), so herbivores must cope with a much longer dry season now than they experienced in 2000.
African landscapes are always changing, and sometimes in unpredictable ways—localised thunderstorms at the beginning of the rainy season can rapidly change a dry dustbowl landscape into green grazing. These “wet footprints” can cover areas as small as 1 km × 3 km; in contrast, a large frontal weather system can cover dozens or even hundreds of kilometres. An ungulate looking for green grazing not too far from drinking water, while trying to avoid predators and hunters, needs the ability to detect such green patches, and the strength and knowledge to move there. Obtaining this knowledge from an ungulate’s perspective of the landscape is no small task. Hopcraft et al. (2014) show how common wildebeest and plains zebra in the Serengeti have different migration strategies: wildebeest move to green grass as quickly as they can, with little effort to avoid predators, whereas zebra gauge predation risk and forage quality concurrent to their trek.
Our ability to understand ungulate movements and other ecosystem patterns has been greatly enhanced over the last few years, as animal-tracking technology (where GPS positions are logged and stored using radio-frequency tracking, mobile phone networks, satellite systems, or any combination) and the resulting analyses became more sophisticated. It is now possible to distinguish between locations where animals often spend a little time (for example, a preferred shady tree for resting during the heat of the day), or the same location where they infrequently spend a lot of time (for example, once during an oestrus cycle, a lactating lioness will spend a lot of time at her den, with cubs). It is also possible to “see” when such an animal changes “mode” of movement. For example, researchers have found that springbok (Antidorcas marsupialis, LC) rams around the Etosha pan in Namibia show sedentary behaviour (small movements around a specific area) when grazing is good, which transitions to searching behaviour during dry seasons (long, relatively fast movements in a straight line) as they move to areas that had water or grass during previous drought periods (Lyons et al., 2013).
If we combine this animal tracking capability with regular remotely sensed images of vegetation quality, then we can start to answer questions about why certain animals move to certain areas. It also highlights the fact that it is very seldom good enough to put a fence around an area and call it preserved. Due to the changing nature of Africa’s savannahs, animals need to be able to move to areas with water or forage, often outside reserves, when they become available. In the face of a changing climate and changing rainfall patterns, this ability to move long distance to reach vital resources remains one of the best adaptation responses that African ungulates may have to cope with climate change. However, fences, roads, and farmland may block these migrations across the landscape, putting those populations at risk of extinction (Section 5.1.1).
African conservation areas need to make provision for these animal movements, or risk conservation areas without sustainable animal populations. This presents a problem—how do we investigate options for large animals to move across a transformed rural landscape and minimise human-wildlife conflict while, on the other hand, still providing access to green grass or drinking water at a specific protected area? Both resources change in location and time of year, and only regular, detailed remote sensing of vegetation, combined with detailed animal movement studies, will provide the necessary picture in time and space.
The popularity and utility of these products have preceded and facilitated the expansion of other remote sensing applications in ecosystem monitoring. Among the most popular are radar products (Figure 10.4), which have become the standard method for obtaining elevation and other terrain data (NASA, 2009, 2013), as well as estimates of carbon stocks (Carreiras et al., 2012). Advances have also been made in using hyper-spectral imagery to monitor soil properties (Mashimbye et al., 2012) and even individual trees (Naidoo et al., 2012). LiDAR has enabled biologists to map three-dimensional vegetation, which can be used to explain animal movements (Loarie et al., 2013; Davies et al., 2016) and measure carbon stocks and forest loss (Burton et al., 2017).
Conservation biologists are often faced with the shifting baseline syndrome, where the reference points they use to measure their progress may be vastly different from earlier states.
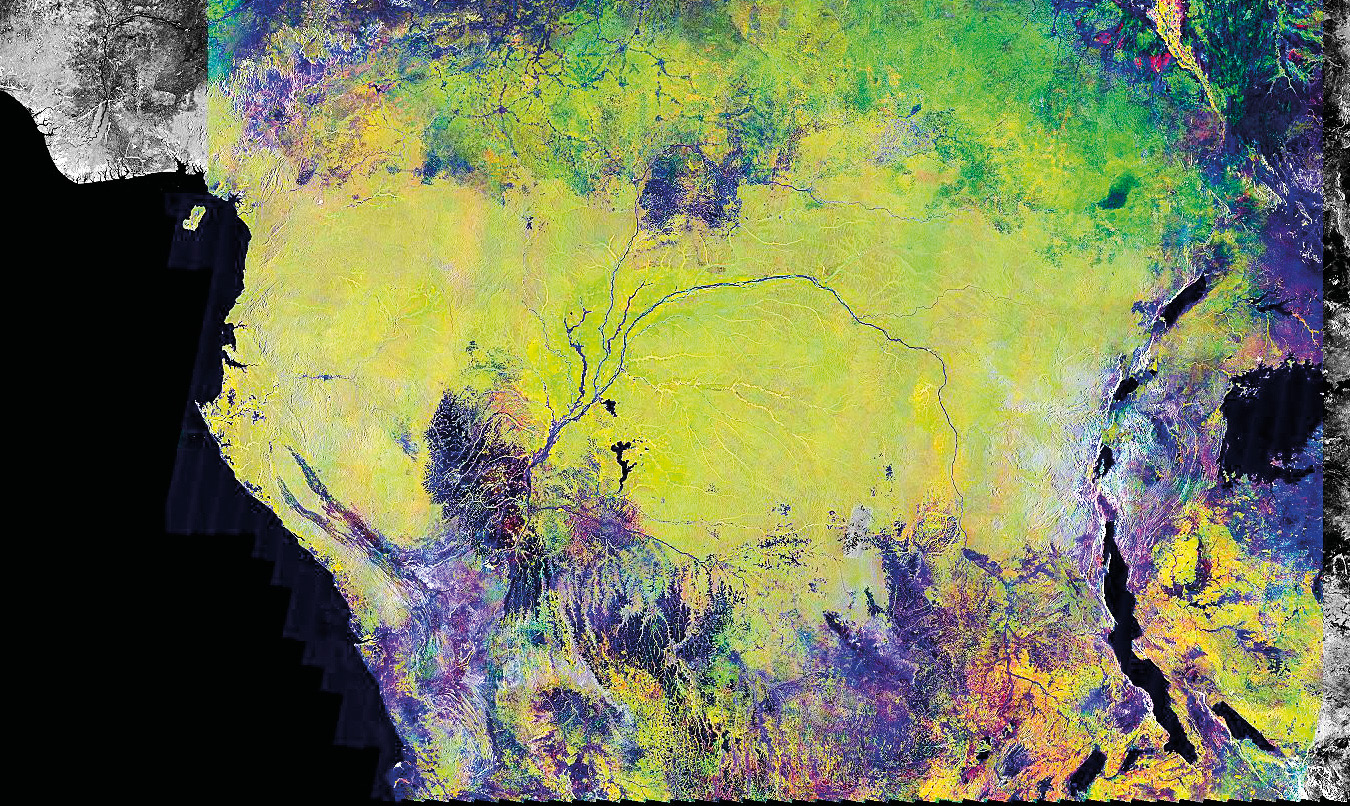
Figure 10.4 A radar composite of equatorial Africa obtained in 1996 by Japan’s Earth Resources Satellite. The area shown covers about 7.4 million km2. Due to the filters applied to the image, yellow represents flooded forests, palm plantations, and urban areas; green represents forest, and black represents surface water. Savannahs may be black, blue, purple, or green, depending on the ecoregion type. Displays such as these allow researchers to map ecosystems, and ecosystem loss at a much larger scale than if only field observations were used. Image courtesy of NASA, https://images.nasa.gov/details-PIA01348.html, CC0.
Despite the opportunities presented by remote sensing, it is critical to remember that it is not a substitute for traditional field monitoring methods. Most importantly, remote sensing applications cannot be considered reliable without verification using field data (see Burton et al., 2017). Most remote sensing products are also relatively new, which does not allow for enough opportunities to compare across time. In the absence of historical remotely sensed data, geospatial analysts usually choose the best reference site currently available; this may expose those analysts to shifting baseline syndrome, because the chosen reference site may be vastly different from earlier states the scientists are actually interested in studying (Bunce et al., 2008; Papworth et al., 2009). Remote sensing is, therefore, not a cure-all for ecosystem monitoring challenges; it is simply a powerful tool to supplement traditional field-based monitoring.
10.2 Maintaining Complex and Adaptive Ecosystems
Even when monitoring data show that an ecosystem is healthy, management is often required to maintain those desired conditions. That is because very few ecosystems are completely free of human influences. For example, rivers and streams carry pollutants far beyond the point of contamination (Section 7.1) and roads acting as firebreaks suppress natural fire regimes. Today, even the most isolated patches of habitats may not be completely protected from the influences of global processes such as climate change. To maintain complex and adaptive ecosystems, conservation biology is guided by four complementary management principles: (1) maintain ecosystem processes, (2) minimise external threats, (3) be adaptive, and (4) be minimally intrusive.
10.2.1 Maintaining critical ecosystem processes
Ecologists generally divide ecosystem processes into four disparate yet interdependent categories: water cycling, nutrient cycling (which include the carbon and nitrogen cycle), energy flow, and community dynamics. The linkages between these processes create feedback loops, where changes in one factor may be amplified elsewhere. Maintaining ecosystem processes is thus very important because small, seemingly small, changes can have major impacts on biological communities.
The water cycle
The water cycle refers to the distribution of water through an ecosystem, and includes the absorption and distribution of water vapour, rainwater, and surface water in lakes, rivers, and oceans. Since much of the water cycle happens out of sight and is generally associated with large-scale phenomena, such as weather patterns and anthropogenic climate change, land managers sometimes fail to recognise how local factors influence the water cycle. This is a grave mistake; many deadly ecological disasters (e.g. desertification, flooding, and landslides) can be attributed to disturbances to the water cycle at the local scale.
Outside of ensuring sustainable use of water resources, maintaining vegetation cover arguably plays the most important role in preserving the water cycle at local scales. Plants and their roots enable soil to store and release water, and make these water reserves available for soil organisms, which in turn aid in decomposition of dead plants and animals (Section 4.2.2). In contrast, a loss of vegetation cover increases surface runoff, which leads to deteriorating soil conditions through nutrient leaching and erosion of fertile topsoil. An increasing number of studies have also shown how forest loss can change a region’s climate by reducing rainfall which in turn exacerbates drought conditions (Lawrence and Vandecar, 2015). For example, forest clearing for agriculture has reduced rainfall by 50% over much of West Africa (Garcia-Carreras and Parker, 2011). Many forest restoration programmes thus focus on reversing these losses.
Complex and adaptive ecosystems provide more opportunities for people to benefit from ecosystem services than uniform plantations with single species
When restoring degraded forests and other ecosystems to repair the water cycle and other ecosystem services, it is important to remember that complex ecosystems with locally-adapted plants are generally the most effective in maintaining the water cycle and other ecosystem services (Burton et al., 2017). There have been cases across Africa where well-intended restoration efforts used fast-growing timber species such as gum (Eucalyptus spp.) and pine (Pinus spp.). While these single-crop plantations may superficially resemble a forest in structure and may even provide some of the same ecosystem services as native plants, some of these fast-growing exotic plants also bring significant environmental harm and negative externalities passed onto local people (van Wilgen and Richardson, 2014). Of particular concern is their role in disrupting local water cycles (Section 7.4.2), ironically the very aspect these forest restoration efforts aim to rehabilitate. The choice of species used for restoration should this be carefully considered to avoid unintended consequences later on.
The nutrient cycle
The nutrient cycle involves the cycling of essential nutrients such as carbon, nitrogen, sulphur, and phosphorus through the ecosystem. Like the water cycle, natural vegetation cover plays an important role in maintaining the nutrient cycle. That is because plant roots slow water runoff which, in turn, help soil to retain nutrients dissolved in water. Plants also form a major component of above-ground and below-ground biomass. When dead plant biomass is decomposed along with animal waste products, nutrients previously absorbed through plant roots are released back into the soil and water, where they can once again be absorbed by living plants and other consumers.
Unfortunately, vegetation cover, decomposition, and fire dynamics (discussed below) alone cannot ensure a healthy nutrient cycle. Much of Africa is nutrient impoverished because soil nutrients are lost quicker than they are replaced. One of the main causes is unsustainable agricultural practices (Sanchez, 2010), such as farming on sandy soils and in tropical forests. These areas are nutrient-poor, so crop yields are typically low. Because these areas are prone to leaching, a large proportion of synthetic fertilisers added to supplement impoverished soils leaches into groundwater or washes into nearby streams and lakes, threatening water supplies by causing harmful algae blooms and eutrophication (Section 7.1.1). To compensate, the failing farmers may resort to even more unsustainable land conversions (Wallenfang et al., 2015). Careful management of the nutrient cycle is thus critical for both biodiversity conservation and socio-economic well-being, particularly given its importance to food security (Drechsel et al., 2001). To achieve this, there is an urgent need to adopt more sustainable land management practices (Chapter 14).
Ecosystem processes are linked into multiple feedback loops, so changes in one factor are amplified elsewhere.
The energy cycle
Energy flow—a crucial component of ecosystem productivity (Section 4.2.2)—refers to the capture and storage of solar energy by primary producers (photosynthetic plants, algae, and some bacteria), and the distribution of that energy to consumers, detritivores, and decomposers. Although solar energy can appear as an unlimited resource in many ecosystems, the energy available to consumers (i.e. herbivores and carnivores) is limited because only about 10% of the energy obtained at one trophic level is passed on to the next (Figure 10.5). Being at the top of the food chain, apex predators are in a particularly vulnerable position because seemingly small disruptions at lower trophic levels will have a cumulative impact on the energy available to them. Such disruptions may include reduced prey populations (e.g. overharvesting of herbivores, Section 7.2) or foraging disruptions (e.g. a predator needing to walk further to find prey). Research from Southern Africa’s Kalahari Desert has showed that such disruptions, which are amplified by predators’ high-energy lifestyles (it takes a lot of energy to bring down a large ungulate!), may put apex predators such as cheetahs on a downward spiral of energy deficits (Scantlebury et al., 2014). While such impacts may not always lead to direct mortality, these insidious, subtle, and easily-overlooked sublethal impacts compromise individuals’ ability to reproduce, with extinction being the end result. To avoid such a scenario, maintaining energy flow generally involves maintaining complex, species-rich ecosystems so that consumers have ample opportunities to fulfil their energy needs for finding prey, growth, reproduction, and other activities.
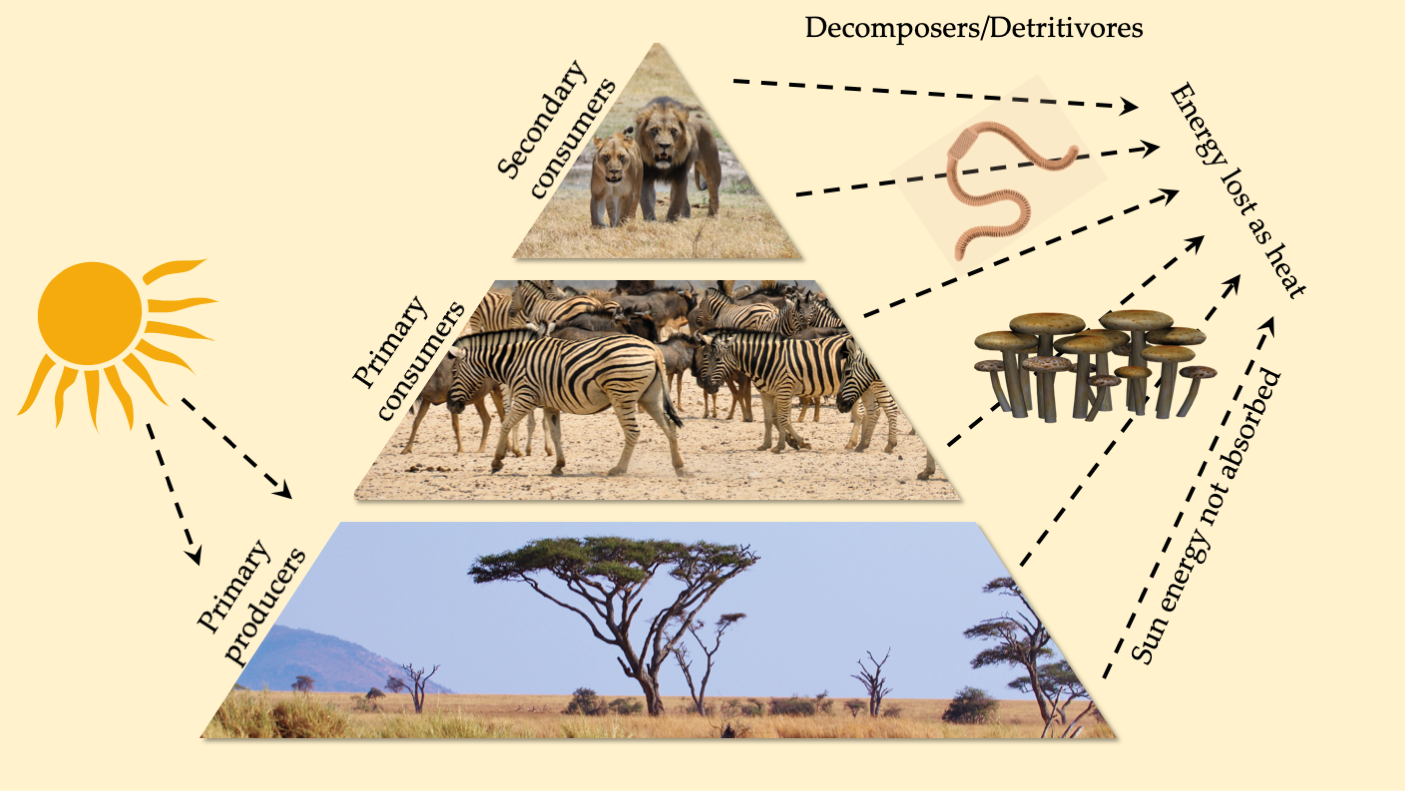
Figure 10.5 A food pyramid of a model savannah ecosystem, showing the various trophic levels and energy pathways. About 90% of energy is lost at each trophic level through respiration and animal waste excretion.CC BY 4.0.
Community dynamics
In ecosystem conservation, maintaining viable populations of different interacting species is as important as maintaining important ecosystem processes, such as ecosystem productivity and ecological succession. This focus often falls on maintaining populations that form part of important mutualistic relationships such as pollination and seed dispersal (Section 4.2.5), predator-prey interactions, and even healthy levels of competitive and parasitic interactions (which allow more species to persist). Of interest is the preservation of keystone species and ecosystem engineers, which has an outsized effect on community dynamics (Section 4.2.1). As illustrated in this, and other, chapters, disrupting community dynamics through pollution, overharvesting, or any other threat facing biodiversity (Chapter 5–7), generally leads to impoverished natural communities. Impoverished communities may in turn provide opportunities for invasive species to colonise an area, further perpetuating biodiversity losses. In Chapter 11, we discuss further how populations and species can be maintained.
Fire Dynamics
Although fire is generally not considered one of the four fundamental ecosystem processes, it plays such an important role in African biodiversity management, including maintaining the four fundamental ecosystem processes, that it deserves its own discussion. African farmers understand the importance of setting fire to keep cropland and grazing pastures productive; burning existing vegetation releases carbon and other essential nutrients beneficial for plant growth into the environment. Similarly, fire also plays a critical role in the flow of energy, community dynamics, and overall maintenance of fire-dependent ecosystems, such as grasslands, savannahs, and Mediterranean communities. Suitably low intensity fires seldom kill living plants; rather, they encourage seed germination and seedling growth by reducing dead material that may crowd new growth, by exposing bare mineral soil (the substrate required for many seeds to germinate), and by releasing vital nutrients into the soil. This periodic removal of dead material also prevents fuel load accumulation, thereby preventing future fires from becoming destructive. In contrast, without fire, fire-dependent ecosystems will slowly transform into unproductive scrublands suffocated by encroaching woody vegetation (Smit and Prins, 2015). Then, when wildfires do occur (e.g. through human negligence or lightning) the resultant accumulated fuel loads increase the intensity and heat of fires, creating very dangerous and difficult to control scenarios.
Obviously, given the potentially destructive force of fire, land managers who use fire as a management tool must consider many aspects before setting a prescribed burn, also known as a controlled burn. Foremost, to prevent a fire from becoming destructive to natural communities and nearby human developments, burning must be done in a well-planned manner with careful consideration given to the area’s ecology, weather forecasts, and fire-readiness of the site (Goldammer and de Ronde, 2004; Kelly and Brotons, 2017). It is also recommended that prior to burning (or any other conspicuous management operation for that matter), land managers develop a public outreach plan to explain to local people the importance of fire in ecosystems management, and the steps taken to keep them and their properties safe. To further improve community relations and education, South Africa’s Working on Fire programme (Figure 10.6) provides scholarships, fire training, and employment opportunities to local youths.
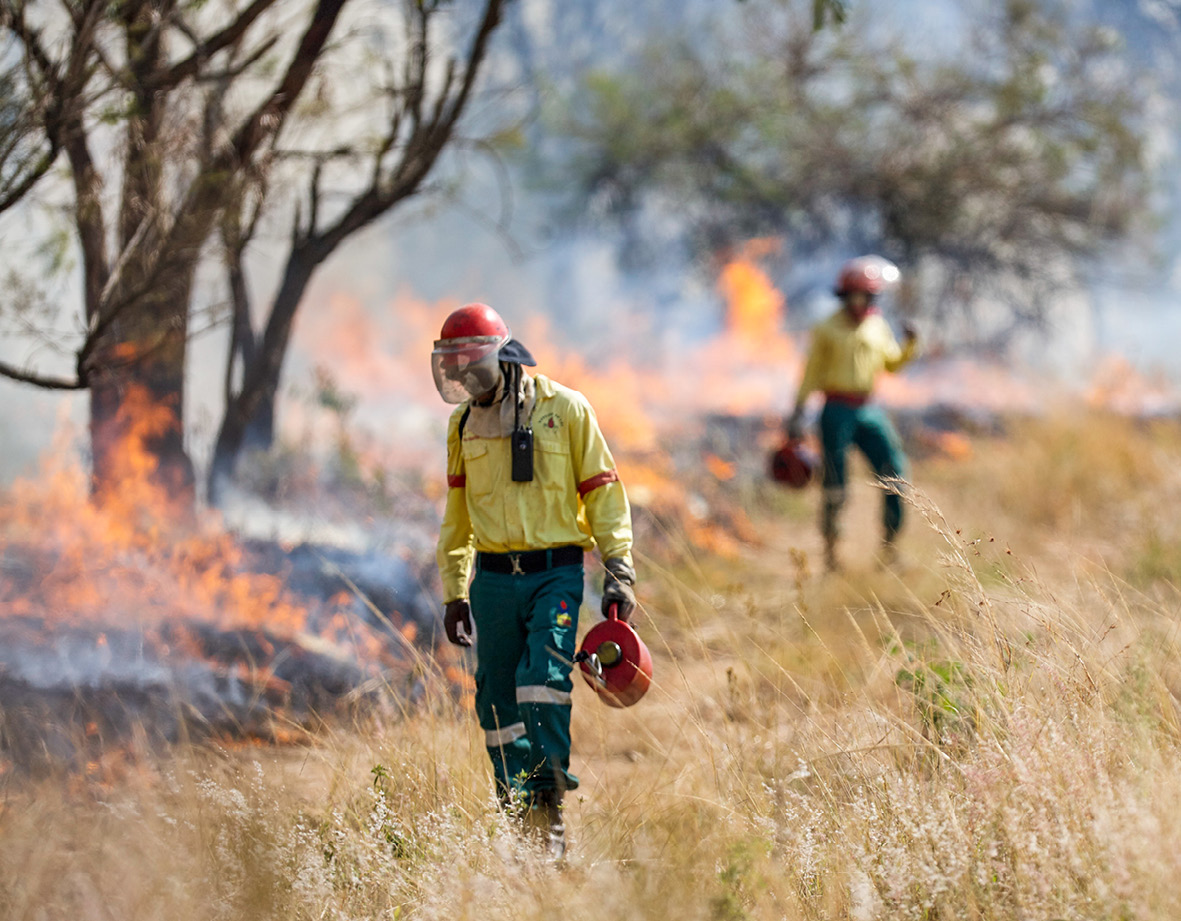
Figure 10.6 A fire crew from South Africa’s Working on Fire programme keeps a close eye on a controlled fire set to keeps the native savannah ecosystem healthy. Photograph by Working on Fire, CC BY 4.0.
Fire management plans that match natural fire regimes produce the best results for effective ecosystem management. Land managers accomplish this by ensuring that their burn plans mimic the local area’s natural fire season, fire frequency, and flame intensity, while also accounting for management goals and local ecological factors such as rainfall and geology (see e.g. van Wilgen et al., 2010, 2014). The size of each burn area must also be considered. Best practices suggest not burning the entirety of a community at a time; rather, burning only portions of an area allows for more habitat heterogeneity, provides opportunities for non-burrowing animals to take refuge in unburned areas, and maximises ecosystem diversity. Bringing all of these aspects together, scientists working in Tanzania’s Ngorongoro Conservation Area determined that the area would respond best if land managers burn up to 20% of their grasslands annually or biannually (Estes et al., 2006). Similarly, field experiments in certain South African grasslands have found that plant diversity is highest when burns occur every second year, in winter or autumn (Uys et al., 2004). Other ecosystems, such as the Cape Floristic Region’s fynbos, may need to burn only once every decade (Kraaij et al., 2013). However, because of the high density of houses in some fynbos ecosystems, the periodic fires needed for locally-adapted vegetation to persist are often extinguished because of the threat to human settlement (van Wilgen et al., 2012).
While fire plays an important role in many African landscapes, it is important to note that overly frequent fires can be a threat even to fire-dependent communities. For example, habitat degradation resulting from too many fires in quick succession can leave a natural community vulnerable to invasions by harmful species (Masocha et al., 2011). Overly frequent fires can also prevent seedling recruitment by directly killing vulnerable young plants, and by depleting the seed bank because seedlings do not have sufficient time to mature and set seed.
Fire-sensitive ecosystems (e.g. tropical forests, high mountains, and peat bogs) must also be managed carefully to avoid fire disturbance, which can lead to habitat loss and edge effects (Chapter 5). One way to accomplish this is to educate farmers living adjacent to fire-sensitive ecosystems on how to safely manage their land with fire. Conservationists also need to be considerate when managing fire-dependent ecosystems adjacent to fire-sensitive ecosystems, as is the case with the unique patches of fire-dependent savannahs—remnants from the last Ice Age 15,000 years ago—that are surrounded by forest within Gabon’s Lobé National Park (Jeffery et al., 2014). Careful fire management, led by good science, is bound to become increasingly important in the future, given that wildfires are expected to become more frequent and more intense under climate change (Pricope and Binford, 2012).
10.2.2 Minimising external threats
Human activity cannot and does not need to be eliminated from nature; in fact, the structure and diversity of many of today’s natural landscapes—and to which today’s wildlife are adapted to—are in part the result of past human activities (e.g. Garcin et al., 2018). Today, there are over 7 billion people on Earth, so our impacts are more pervasive than for the majority of history. There is, thus, an urgent need to utilise natural resources in such a way that future generations will also benefit from the ecosystem services that previous generations have left us. This requires a concerted effort from every sector in human society to minimise those threats we impose on the ecosystems around us. This includes preventing pollution, large-scale human disturbances, overharvesting, and habitat destruction (Chapter 5–7).
Major strides have been made in recent years towards achieving these goals. Governments are updating laws to safeguard the environment, industries are refining recycling and waste disposal methods, new techniques are being developed to remove pollutants from the environment, and individual citizens are becoming more aware of their individually small but collectively significant impacts on the environment. We should be proud of the progress being made and continue to strive for improvements. But one external threat that requires greater attention and understanding is invasive species.
Controlling invasive species
Invasive species degrade and destroy natural ecosystems by outcompeting native species, disturbing ecosystem processes, and altering the physical environment (Section 7.4). Limiting these harmful impacts can be particularly challenging since exotic species that establish themselves in a new area can build up such large numbers, become so widely dispersed, and be so thoroughly integrated into ecosystems (i.e. naturalised) that eradicating them entirely would be extraordinarily difficult and expensive, or as in the case of tickberry (Lantana camara) perhaps even impossible (Bhagwat et al., 2012). This is not only a problem facing conservation biology, but also agriculture, where invasive species often spread from one farm to another, forestry, where invasive species are spread between saw mills and along logging routes, and fisheries, where native resources are outcompeted, sometimes up-ending an entire local industry. The impact of invasive species on farming communities is particularly severe—they lose tens of billions of dollars each year while trying to combat deteriorating grazing lands, reduced crop yields, and escalating pest control expenses. One study from South Africa calculated that invasive plants result in financial losses of US $646 million each year—this figure would have been US $5 billion if invasive species control measures already implemented were absent (de Lange and van Wilgen, 2010). Consequently, a range of stakeholders have invested considerable resources in combatting invasive species.
The most important step in preventing biological invasions is to prevent the initial establishment of problem species. This requires educating people about the dangers posed by invasive species.
Because invasive species are often very hard to eradicate once established (Figure 10.7), the foremost step in avoiding invasive species’ harmful impacts is to avoid opportunities for new invasions (Section 7.4.1). This requires raising awareness across all levels of society about the dangers posed by invasive species, both to the natural world and to agricultural and natural resources systems. There is also a need for citizens, scientists, and industry to monitor for potential and known invasive species, and promptly implement intensive control efforts to stop establishment and spread. The Global Register of Introduced and Invasive Species (http://www.griis.org) is a free, online searchable source to facilitate these tasks, by providing information about the impact and control of invasive species. Governments can also partake in efforts to control invasive species. While most African countries screen agricultural imports for pests, countries, such as Australia and New Zealand, take this task particularly seriously, with trained officials screening each visitor (and returning residents) and package for hitchhiking species before they cross those countries’ borders. Lastly, it would require increased dialogue between conservation biologists and land managers to make a careful and thorough assessment prior to the deliberate introduction of a new species, even if thought of as beneficial.
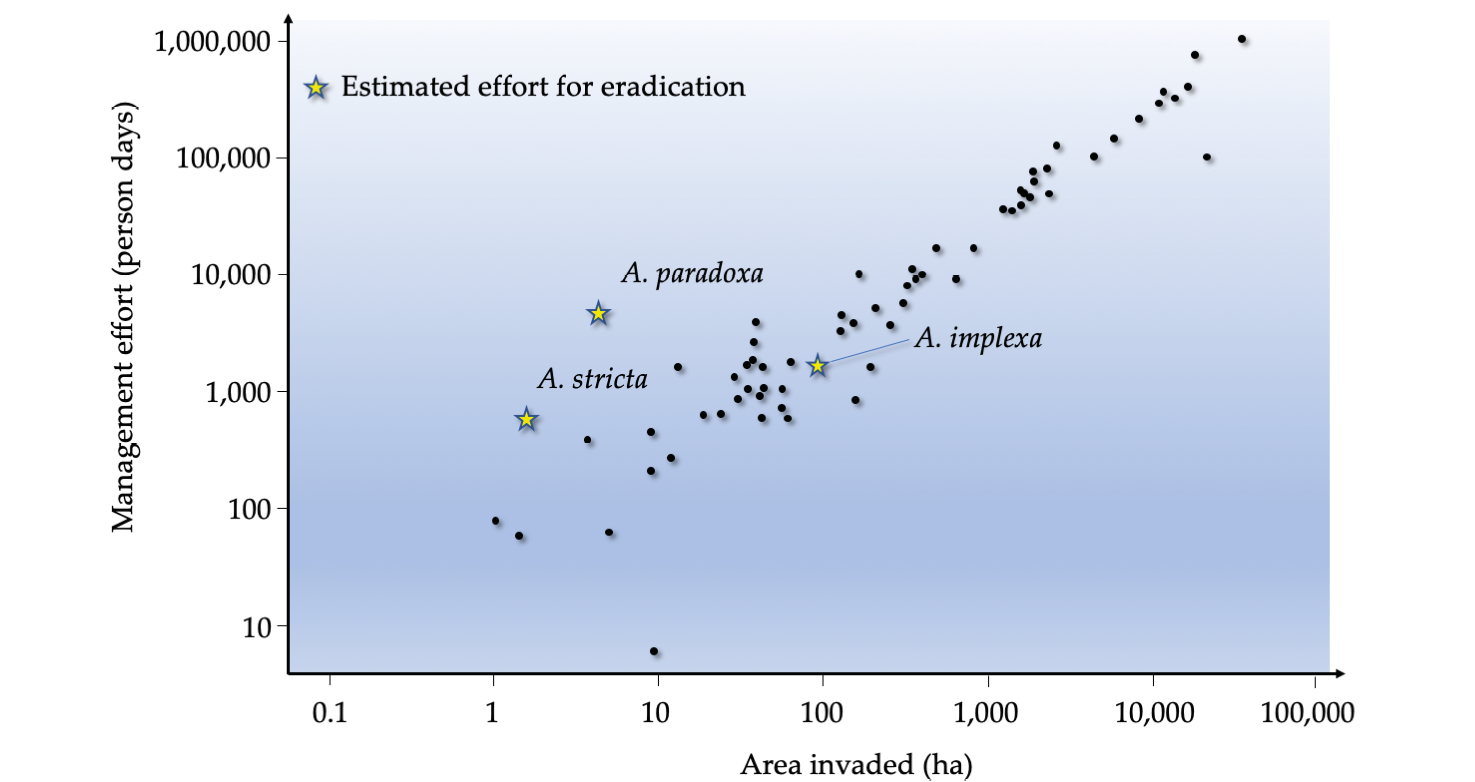
Figure 10.7 Data on the control of invasive species in South Africa have shown that the larger the infestation (a function of time passed since establishment), the more resources are required for eradication. For that reason, it is critical to avoid establishment of invasive species in the first place, and to respond promptly once new invasions have been discovered. Also shown is the effort required to eradicate three species of Australian wattle (Acacia spp.), based on extent of invasion. After Wilson et al., 2013, CC BY 4.0.
Despite best practices, not all invasions can be prevented, and for those that do occur, an early detection and rapid response strategy offers the best chance to limit harm. This usually involves raising awareness of potential invasive species to ensure biologists and other stakeholders will recognise a new invasion, efforts to screen for such species on a regular basis, and implementing direct attack approaches, such as using herbicides, pesticides, or mechanical control once detected. While addressing a new invasion as soon as possible, it is also important to consider and contain the risks each direct attack approach carries. For example, herbicides and pesticides carry a risk of killing non-target native species via pesticide drift (Section 7.1), while mechanical control may cause disturbances such as trampling, undue soil disturbance, and even pollution.
Controlling invasive species that have become established will require substantially more resources and manpower to combat than those detected early on. Even so, it is still worth initiating control measures as early as possible since harm and resource needs will only escalate over time. To overcome these impediments to invasive species control, the South African government established an exemplary model, the Working for Water programme, in 1995. Working for Water has three goals—poverty relief, water conservation, and invasive species control—which it accomplishes by combining invasive species control with job creation and social upliftment (van Wilgen and Wannenburgh, 2016). Specifically, the programme hires and trains unemployed people to eradicate water-thirsty invasive shrubs and trees across South Africa; some of the removed plants are subsequently sold as firewood at local markets at a profit for the participants. In its first 20 years, the programme has created over 227,000 person years of employment and treated over 28,000 km2 of invasive species (Figure 10.8). Hopefully programmes, such as these, will inspire more governments to act to restore degraded ecosystems to their previously healthy state.
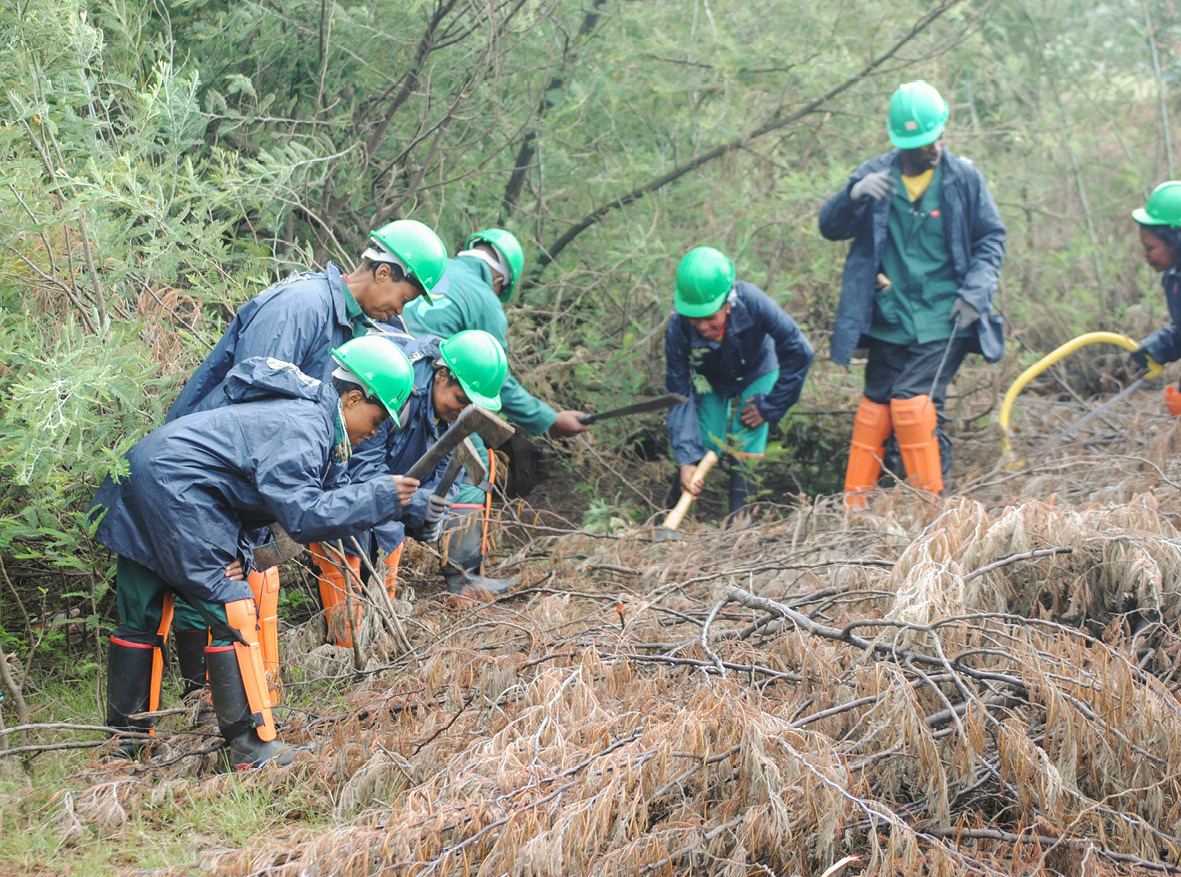
Figure 10.8 Members of South Africa’s Working for Water programme mechanically removing invasive Australian wattles (Acacia spp.). To make sure the wattles don’t grow again, the stumps are also treated with a herbicide. Photograph by Christo Marais/Department of Environmental Affairs, CC BY 4.0.
Another method to manage established pests is biological control, also called biocontrol. Biocontrol typically relies on one or more natural enemies from an invasive species’ original range to control the pest in its introduced range (Section 4.2.7). One of the main benefits of biocontrol is that it ensures cost-effective, long-term, area-wide control of an invasive species, beyond the capabilities of chemical pesticides and mechanical control. Biocontrol also allows for opportunities to control invasive species that are hard to manage with chemical pesticides and mechanical control (at least without significant additional harm to the environment), such as submerged aquatic weeds (Coetzee et al., 2011). Third, biocontrol agents are highly host-specific, thereby eliminating the impact that chemical pesticides and mechanical control have on non-target organisms. Lastly, an effective biocontrol agent ideally eliminates the need for chemical pesticides and mechanical control, thereby reducing threats such as pesticide pollution (Section 7.1) and ecosystem degradation (Section 5.1).
Biocontrol does have some drawbacks. Primary among them is the significant upfront investment required, as candidate species first need to be found, and then extensively tested for host specificity and potential interactions with native wildlife before being released. Biocontrol also requires careful monitoring after release to determine effectiveness as well as to carefully check for impacts on non-target native species. This monitoring needs to be conducted over the long term, because biocontrol agents typically require several years before they establish self-sufficient colonies in the wild and might only then show signs of unintended impacts. Because alternative methods for controlling invasive species can also kill biocontrol agents (causing conflicting results and wasted resources), additional coordination is required before applying biocontrol and alternative pest management strategies simultaneously in the same area. Lastly, there is no guarantee that a biocontrol agent will be effective. For example, the tickberry continues to thrive despite the release of over 40 biocontrol agents (Zalucki et al., 2007). But when successful, the long-term savings from these upfront investments are generally well worth it. One study in Benin found that biocontrol of water hyacinth required a US $2 million upfront investment, but the resultant water quality improvements increased local incomes by US $84 million per year (de Groote et al., 2003). Another study from South Africa estimated a net gain of 50–3,500 times the investment, depending on the specific biocontrol agent used (de Lange and van Wilgen, 2010).
Several very successful biological control programmes have been implemented in Africa over the past century. Most famous is the rescue of the cassava crop (see Box 4.3). Another successful biocontrol programme, implemented across much of the continent, has reduced Kariba weed (Salvinia molesta) by over 95% within just a few years (e.g. Mbati and Neuenschwander, 2005; Diop and Hill, 2009; Martin et al, 2018). South Africa has been particularly active in the research and introduction of biocontrol agents. From 1913, when South Africa started controlling invasive cacti with hemipterans, to 2017, a total of 93 biocontrol agents have been released for the control of 59 invasive species (Zachariades et al., 2017).
While the most popular biocontrol agents generally involve insects, disease-causing pathogens can also be used for biocontrol. Feline panleukopenia virus (also known as feline distemper) was highly effective in managing a feral cat population that caused the extirpation of seabirds on Sub-Antarctic Marion Island; some birds are now even returning as breeders (Bester et al., 2002). In an effort to reduce the use of chemical pesticides on food crops, efforts are also currently underway to find fungal pathogens to control introduced pests impacting African crops, including pea leafminers (Liriomyza huidobrensis), originally from South America (Akutse et al., 2013), and banana weevils (Cosmopolites sordidus), originally from Southeast Asia (Akello et al., 2008).
The most effective pest control programmes use an integrated pest management (IPM) approach that relies on using multiple control methods either simultaneously or in succession.
Some of the most effective pest control programmes use an integrated pest management (IPM) approach that relies on using multiple pest control methods described above either simultaneously or in succession (van Wyk and van Wilgen, 2002). Strategic planning to coordinate best practices can also help offset some of the costs of invasive species control (Rahlao et al., 2010) and ensure that important pest sources are not missed (van Wilgen et al., 2007). When considering the best method to control an invasive species, it may also help to consider how our own actions inadvertently encourage invasive species. For example, an over-reliance on synthetic fertiliser has been shown to cause eutrophication (Chislock et al., 2013) and encourage growth of aquatic invasive plants (Coetzee and Hill, 2012; Bownes et al., 2012).
Even though the impacts of invasive and other exotic species are generally considered negative, they do occasionally provide some benefits. For example, Australian pines (Casuarina equisetifolia) have been planted widely throughout Africa for timber, charcoal, and to stabilise eroding lands. Some people harvest invasive species to eat or to sell; examples include water hyacinth (Figure 10.9), prickly pears (Opuntia spp.), and Mediterranean mussels (Mytilus galloprovincialis). The latter has also become an important food source for the African black oystercatcher (Haematopus moquini, NT) (Kohler et al., 2009). Similarly, nearly 18 species of diurnal raptors, including four globally threatened species, nest and roost—sometimes in colonies numbering thousands of individuals—in stands of invasive Australian gum (Eucalyptus spp.) trees (Allan et al., 1997; Jenkins, 2005). Many birds also favour fruits produced by invasive plants, such as the tickberry and syringa (Melia azedarach); this however allows those plants to spread even further. Because they reproduce so fast, water hyacinth has been investigated as a resource for producing bioenergy (Güereña et al., 2015). A ground spider (Prodida stella, CR), endemic to the Seychelles and threatened by sea level rise (Gerlach, 2014), was first described from specimens collected on Australian pine, and may thus persist on trees further inland as their original range is submerged by rising sea levels. Nevertheless, assessments generally show that costs incurred from invasive species outweigh the benefits (Mwangi and Swallow, 2008). It is thus important to consider whether native species can fulfil the same functions in those cases where benefits of invasive species are touted.
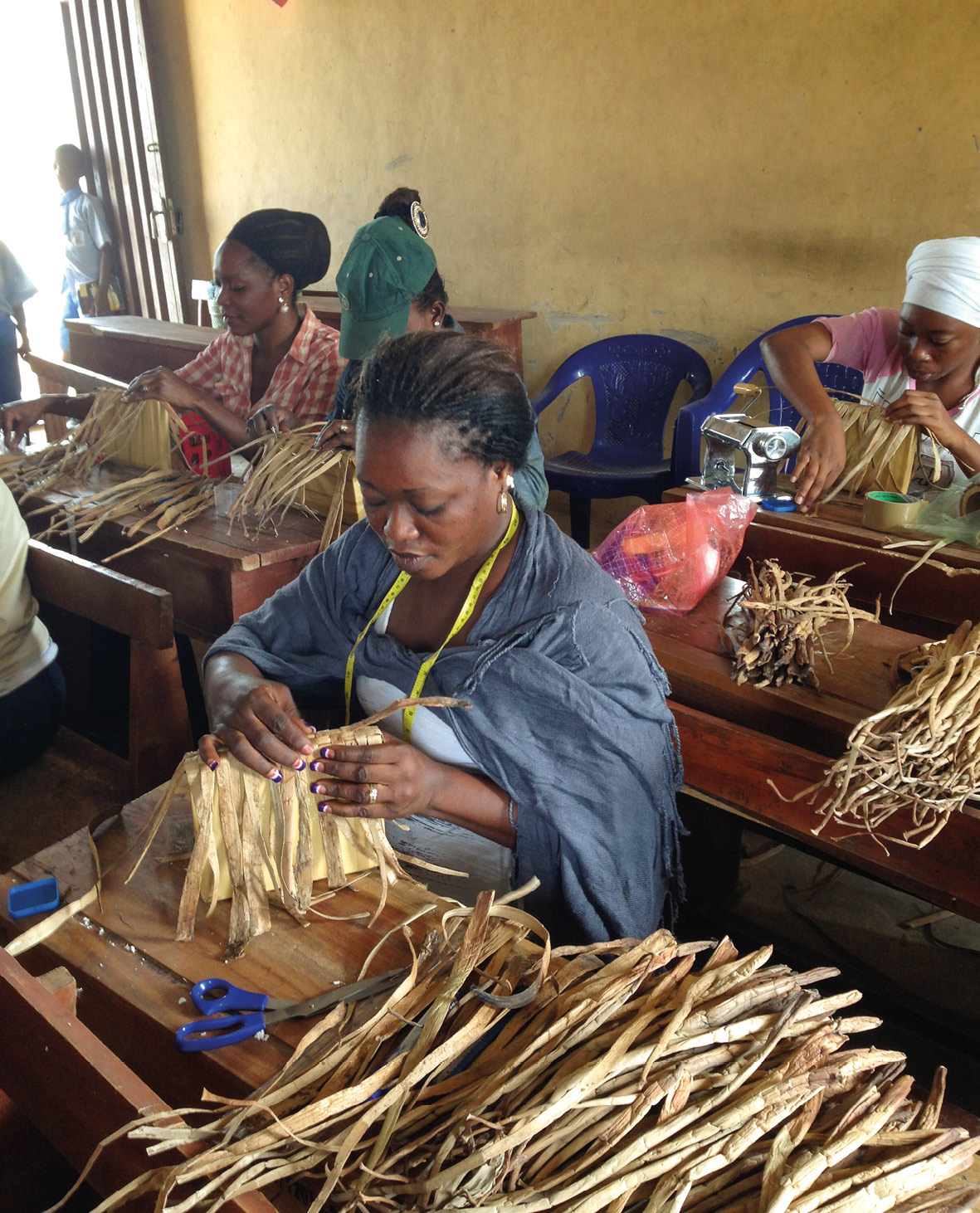
Figure 10.9 Turning an undesirable weed into a cash crop: entrepreneurs from South Africa to Lake Victoria and Nigeria (pictured) have developed alternative income streams by training members of their communities in how to make handcrafts from invasive water hyacinth. Photograph by MitiMeth, CC BY 4.0.
10.2.3 Adaptive management
In decades past, ecosystem management in Africa was generally conducted following a laissez-faire (i.e. hands-off) approach where natural processes were allowed to follow their own course, with interventions only implemented when subjectively deemed absolutely necessary. While this passive management style may have worked in an era when ecosystems were less fragmented by roads and fences, and the impact of pollution and invasive species transported along rivers and streams were limited. But maintaining healthy ecosystems (especially small ones) through limited action is becoming increasingly difficult in today’s human-dominated world. Leaving concerns unattended may seem fine in the short term, but such neglect can create problems that are very difficult to contain later on. For this reason, it is increasingly necessary to actively manage ecosystems not only to achieve conservation goals, but even to just avoid degradation of current conditions.
A major challenge of active (and reactive) conservation management is that nature consists of thousands of interacting components and feedback loops. Because it is impossible to fully understand how these different components interact, management strategies are typically implemented with an incomplete understanding of how interventions may impact broader ecosystem processes.
Management actions that draw from experimentation and prior experiences often deliver the desired results. However, despite good intentions and careful consideration, some interventions may later give rise to a cascade of unintended consequences that run counter to overall conservation goals. South Africa’s Kruger National Park provides a good example. Conservation managers here once thought they could better protect the local wildlife by fencing the Park and constructing artificial waterholes at regular intervals, a policy that was implemented primarily in the 1960s and 1970s (Smith et al., 2007; Venter et al., 2008; van Wilgen and Biggs, 2011). But instead of providing conservation benefits, the fences blocked dispersal routes, which caused grazing herbivores to be increasingly sedentary around waterholes, leading to overgrazing. The increased availability of surface water also allowed elephant populations to increase to a point where they became a threat to other taxa, particularly large fruit-bearing trees such as baobabs (Adansonia digitata). The construction of artificial waterholes in arid sections of the Park saw an expansion of plains zebra (Equus quagga, NT) and common wildebeest (Connochaetes taurinus, LC) distributions, which in turn also attracted more lions into these arid areas. This increasing grazing competition and predation pressure caused populations of four locally rare antelopes to fall by 73–88% between 1986 and 2006, prompting fears of imminent extirpations of these iconic species in one of Africa’s premier protected areas.
Despite good intentions, conservation activities may give rise to unintended consequences that run counter to overall conservation goals. Adaptive management can reduce further harm.
While it is hard to escape the looming threat of unintended consequences harming biodiversity, their impacts can be mitigated. One of the first steps involves setting management goals and objectives through close working relationships with a wide variety of stakeholders, including local people (Box 10.3) and scientists who can provide additional insights into the local social-ecological context within which land managers operate. Also important is developing a monitoring protocol that enables land managers to assess whether conservation goals are being met. When monitoring shows that management actions are ineffective or detrimental, it is absolutely critical to be willing and able to integrate this improved understanding into revised management strategies. This process, whereby new knowledge gained through repeated cycles of learning is used to revise and refine conservation strategies and management goals, is known as adaptive management (Venter et al., 2008; van Wilgen and Biggs, 2011). Rather than punishing management errors, adaptive management embraces the idea that we live in a complex world, and that management strategies frequently need be revised to account for new knowledge, shifting priorities, and even evolving societal values. Some of the best adaptive management plans explicitly mandate regular (e.g. every 5 years) reviews, where management strategies, goals, and objectives are formally reviewed and updated.
Box 10.3 Environmental Governance in the Serengeti Ecosystem
College of African Wildlife Management,
Mweka, Tanzania.
akisingo@mwekawildlife.ac.tz
The greater Serengeti ecosystem of Tanzania (Figure 10.C) represents one of the most biologically productive ecoregions in the world. In recognition of its biological importance, much of this ecosystem is safeguarded under a mosaic of government, private, and co-managed protected areas, as well as community conserved areas (Kisingo, 2013a). Among these protected areas is the world-famous Serengeti National Park, as well as the Ngorongoro Conservation Area.
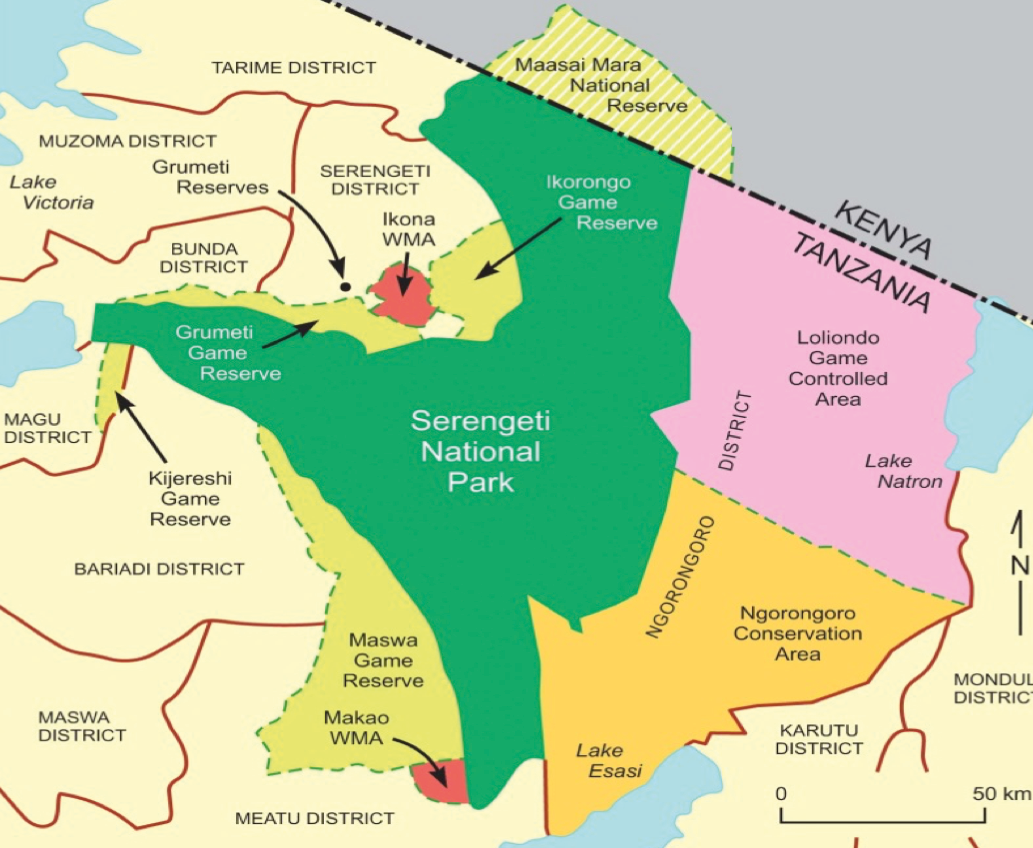
Figure 10.C A map of the greater Serengeti ecosystem, showing the location of the major protected areas in the region. Map by Alex Kisingo, CC BY 4.0.
The various governance models that dictated human actions in the colonial, post-colonial, and contemporary eras have had a profound effect on the ecosystem’s conservation outcomes (Polasky et al., 2008; Sinclair et al., 2008). During most of the colonial period and immediately after Tanzania gained independence in 1961, management of the ecosystem was in the hands of government, which followed a fortress conservation approach (i.e. “fences and fines”). This included evicting traditional peoples from their ancestral lands, restricting the use of wildlife and other natural resources, and imposing heavy penalties on those on the wrong side of wildlife laws. This approach severed the link between people and nature, creating an atmosphere of hostility and resistance to conservation initiatives amongst the local communities. The results have been increased subsistence and commercial poaching, human encroachment into wildlife habitats, and a general lack of community support for conservation (Kisingo 2013b). As a result, Tanzania has seen its wildlife populations reduced and migratory corridors blocked, while invasive plant species are spreading and human-conservation conflicts are intensifying.
In contrast to this counter-productive approach, involving local people in decision making and implementation can enhance attainment of conservation and social outcomes. Such an involvement can occur through training and employment in conservation enterprises, developing social infrastructure through conservation-related financing, and improving a democratic governance space through increased awareness and capacity building (Kisingo 2013a). This socio-economic transformation, where local communities experience the benefits of conservation activities first-hand, can even create a positive feedback loop where local people not only reduce their dependence on protected wildlife resources for survival, but also become inspired to initiate their own grassroots conservation initiatives.
In 1989, nearly 30 years after Tanzania gained independence, some attempts were made to empower local people in the governance of the Serengeti ecosystem. The first major step in this regard involved developing the Serengeti Regional Conservation Strategy (SRCP), which encouraged the creation of integrated conservation and development projects (ICDPs, see Section 14.3) as a means for local people to gain direct benefit from conservation (Kisingo, 2013a). This was followed by the establishment of the Ngorongoro Pastoral Council in 2000, to include pastoralist communities living in the Ngorongoro Conservation Area (NCA) in decision making and protected areas management. Then, in 2003, the Ikona and Makao Community Wildlife Management Areas (WMAs) were established as a first step towards the co-management of wildlife in the Serengeti ecosystem.
Unfortunately, despite good intentions, these initiatives only had a limited impact in empowering local communities. An important reason for this failure was a general incompatibility between local policies and national legislations, notably a legal framework that favoured a top-down approach to decision making over the wishes and values of local communities. In the NCA, communities were more involved in the provisioning of scholarships and socio-economic assistance, in effect surrendering decision making power to government authorities. Local communities thus either never had a real seat at the table during the development and implementation of management strategies, or lost what little they had over time (Kisingo, 2013a). It should thus not come as a surprise that management of the Serengeti ecosystem generally presents mixed results in terms of conservation and social outcomes. Although wildlife populations are still better off in government protected areas when compared to WMAs, neglecting these community conserved areas (which function as critical wildlife corridors) undoubtedly also negatively impacts wildlife in government protected areas.
To be more effective at meeting conservation goals, there is an urgent need to adapt and reengineer ecosystem governance structures in the greater Serengeti ecosystem. Involving more stakeholders, particularly local people, would be an important first step. This involvement should be honest and transparent. Instead of calling one or two community representatives to a workshop to secure a rubber-stamp, start by gathering input from a wide variety of stakeholders and make an effort afterwards to show how that input was considered in final plans. In this way, even those who do not get what they want will at least know that they were heard, and hopefully understand why their desires were not met. There is also an urgent need for capacity building, to develop conservation management expertise among members of local communities, rather than the current over-reliance on outside experts who do not understand local dynamics. This could start small: community members can, for example, be involved in anti-poaching efforts through the use of Village Game Scouts (VGS), a strategy that reduces operational costs in tandem with boosting local incomes. The most promising and eager scouts can then be provided with additional professional development opportunities. Empowering local communities in this way, and enabling them to benefit from conservation activities, will provide opportunities for a wider variety of people, beyond just foreign tourists, to understand how conservation actions can support and benefit livelihoods. This will result in social outcomes that are desired by local communities, and in return, conservation authorities who then gain local support for future actions.
10.2.4 Being minimally intrusive
While adaptive management often necessitates active management, it does not embrace a philosophy of intervention for its own sake. If managers try to maintain their land by intervening in every aspect in nature, conservation will become inhibitively expensive, especially as the costs of managing unintended consequences escalate. Rather, effective adaptive management plans generally embrace a philosophy of “management by exception” (Venter et al. 2008), whereby interventions are implemented only when certain pre-determined thresholds of concern are exceeded, or when monitored trends suggest that those thresholds will soon be exceeded. (For examples of such thresholds, see van Wilgen and Biggs, 2011).
Thinking back to the example from Kruger National Park, discussed above, when it became clear the four species of antelopes were on their way to extirpation, a research programme was initiated to identify the causes for these population declines. Based on this research, park managers decided that the best way forward was to close the majority of the artificial waterpoints, and move some antelopes to a predator-proof enclosure where they can safely breed (van Wilgen and Biggs, 2011). To reverse the damage caused fences, park managers also prioritised re-establishing free movement of animals by removing these fences between the park and some adjacent properties (Venter et al., 2008). While lion and zebra populations subsequently moved out of the Park’s arid region as hoped, and elephant populations stabilised, numbers of the four antelope species remained stubbornly low. This prompted park managers to evaluate whether the resources invested in these locally rare but globally common ungulates could be better spent on globally rare species occurring in the park, such as black rhinoceros (Diceros bicornis, CR). In other words, they adapted their threshold of concern from focussing on locally rare species to prioritising globally rare species (van Wilgen and Biggs, 2011). This decision remains controversial, even among park managers concerned about the potential loss of genetic diversity within the four ungulates. But adaptive management allows for shifting priorities; park managers may very well revisit their decisions if and when the park’s rhinoceros are secured, and funds are freed up for other activities.
10.3 Restoring Damaged Ecosystems
Ecosystems are regularly disturbed by natural phenomena such as floods from cyclones/hurricanes, or wildfires started by lightning. Nevertheless, natural disturbances typically lead to succession and a return to ecological conditions that can sustain high levels of biodiversity. In contrast, ecosystems that humans have damaged or destroyed through activities such as unsustainable agriculture and deforestation, overgrazing, or pollution tend to lose their ability to rebound without human intervention.
Ecological restoration aims to restore damaged ecosystems to a point where their ecosystem functions and species composition resemble their original or near-original state.
Ecological restoration is the practice of restoring damaged ecosystems to a point where their ecosystem functions and species composition resemble their original or near-original state. Restoration ecology, in turn, is the scientific study of restoring damaged ecosystems, communities, and populations. Ecological restoration is often the best method for providing for the long-term use of degraded sites, whether considered from the perspectives of ecology, social benefit, or even economic benefit. Consequently, and unsurprisingly, this practice and the science originally developed in response to attempts to restore economically valuable ecosystem functions: creating wetlands to prevent flooding, reclaiming mining sites to prevent pollution and soil erosion, revegetating overgrazed rangelands to increase grass production, and planting trees on cleared areas to improve agroforestry.
Best practices in ecological restoration have undergone major advances in recent decades. In the past, restoration methods mostly aimed for quick economic benefit, which resulted in simplified ecosystems that either failed to establish or degraded after a short time. To avoid such costly mistakes, restoration plans of today increasingly aim for the permanent re-establishment of healthy ecosystems that could support sustainable industries such as ecotourism, wildlife management, carbon sequestration, and low-level grazing by livestock. Ecological restoration often also makes economic sense; a study from South Africa found that every US $1 invested in restoring ecosystem services would generate US $8.30 for the local economy (de Wit et al., 2008).
Many grassroots conservation groups are at the forefront of initiatives that use ecosystem restoration to help make the connection between healthy ecosystems and socio-economic well-being. One prime example is the Green Belt Movement, a Kenyan initiative led by rural women to combat deforestation and restore degraded forests. They do this by helping rural women work together to grow and plant trees. Since its founding in 1977, the organisation has overseen the planting of over 51 million trees, which has helped restore forests on Mount Kenya, the Aberdares, and the Mau Complex. The planted trees have prevented erosion, stored rainwater, and provided firewood, timber, and food. In addition, over 30,000 women have been trained in sustainable trades such as forestry, beekeeping, and food processing.
10.3.1 Ecological restoration approaches
There are four main approaches to ecological restoration (Figure 10.10):
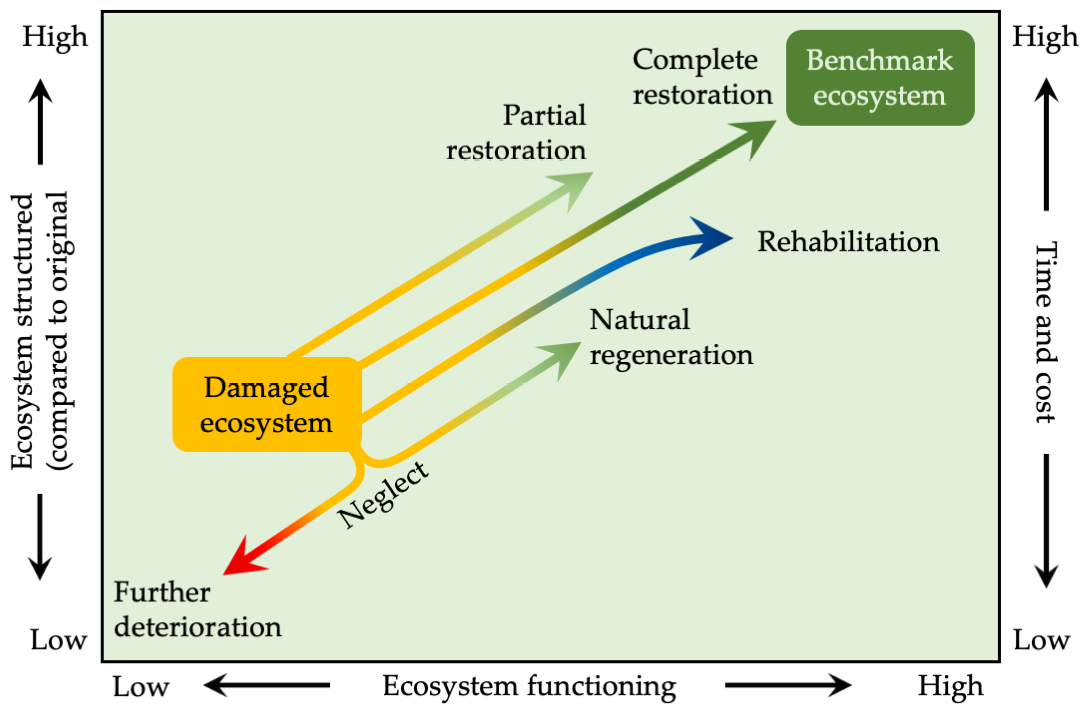
Figure 10.10 Several approaches can be followed when restoring an ecosystem, ranging from taking no action and letting the ecosystem regenerate naturally to completely restoring a degraded site. The best course of action will depend on a project’s end goals and the resources available. After Bradshaw, 1990, CC BY 4.0.
- Natural regeneration. Degraded areas, such as abandoned fields or logged areas, are allowed to naturally reseed and return to grasslands or forests. Land managers often choose this approach when active restoration is too expensive, when earlier restoration attempts have failed, or when experience has shown that the ecosystem is resilient and can recover on its own (e.g. Crouzeilles et al., 2017).
- Rehabilitation. Land managers improve conditions of a degraded ecosystem by transitioning it to another, different ecosystem type. For example, land managers could rehabilitate a degraded forest by transitioning it to a tree plantation. Rehabilitation could involve replacing just a few species or many species.
- Partial restoration. Land managers restore some ecosystem functions and some of the species that were dominant or characteristic of the ecosystem. For example, as a part of a grassland restoration, land managers might initially replant a few key species that are hardy and contribute to ecosystem functioning; they could delay restoration of rare species until later phases.
- Complete restoration. Land managers restore an area to benchmark ecosystem structure, mix of species, and ecosystem functioning. Complete restoration usually requires an active programme to modify the site, reintroduce native species, and eliminate or reduce the factors that were degrading the ecosystem.
Before a restoration project is initiated, and the type of approach is decided upon, land managers must consider how quickly the ecosystem can recover, resource needs and availability, the availability of locally adapted taxa, and the work that might be required to allow the restored community to persist over the long term. Examples of specific considerations include how to prepare soils, how to handle translocated organisms, when and how much fertiliser and water to add, and how to prevent invasions by unwanted species (Galatowitsch and Richardson, 2005; Zabbey and Tanee, 2016). It is also important to remember that ecosystems generally fail to recover if the factor that caused them to become degraded in the first place is not removed or reduced. For instance, efforts to reverse desertification (Section 5.3.4) would require a reduction of grazing pressure and unsustainable agricultural practices.
To measure restoration success, biologists often aim to restore degraded areas to conditions (ecosystem functions or species composition) comparable to a chosen benchmark or reference site. Reference sites provide practical targets for restoration and can be used to quantitatively assess of the success of a restoration project. Comparing restoration progress against a reference site also allows land managers to intervene or adjust their methods if restoration goals are not being met. This approach, in which land managers monitor conditions and adjust their protocols as and when needed, is known as adaptive restoration. (For a general discussion on adaptive management, see Section 10.2.3.)
10.3.2 Major restoration targets
Many human-altered ecosystems in Africa have proven to be good candidates for ecological restoration. These include tropical rainforests, wetlands, rangelands, and coral reefs. In addition, restoration projects in urban areas (Box 10.4) have become popular in recent years in part due to the enhanced quality of life for people living in the area.
Box 10.4 Sustainable Forest Restoration Using Natural Vegetation
School of Biological Sciences, University of Nairobi,
Nairobi, Kenya.
samuel.kiboi@uonbi.ac.ke
Deforestation is one of the main driving forces of biodiversity loss in Africa. Many rural and urban communities rely on wood biomass for energy in the form of either charcoal or firewood. This means that they must continuously source for the firewood or charcoal by harvesting living or dead trees. In many cases, the available energy source is live trees on farmlands which are planted as border trees, or random remnants of pre-existing vegetation within the farm. In some instances, farmers who have land in less densely populated areas have portions of forested areas or woodlots which are under continuous disturbance from wood harvesting. This is more common in rangelands or areas that have lower agricultural productivity. In other areas, such as urban settlements bordering forests, such as Kibera in Nairobi Kenya, there has been extensive harvesting of firewood and sometimes selectively for medicinal purposes or wood carving (Furukawa et. al., 2011).
Given the known benefits of intact forests, including improving food security and climate change mitigation, there are currently several efforts aimed at increasing Kenya’s forest cover both in protected and unprotected areas. The Kenya Forest Service has always been at the forefront of restoration in protected areas, particularly in gazetted forest areas. Despite the general enthusiasm to increase forest cover, many structural and informational challenges remain. Most reforestation programmes classify seedlings as either “exotic” or “indigenous”, but do not consider which species are best suited to local conditions. In addition, despite the numerous reforestation programmes initiated by individuals, government entities, and corporations, there is generally minimal follow-up maintenance after planting, which can jeopardise an entire project. The first three years after planting are especially crucial for proper seedling establishment and require intensive management, including weeding, mulching, and protection from herbivores. Perhaps the biggest challenge to the sustainability of these reforestation initiatives is the slow growth rate of many valuable indigenous trees that does not meet short-term harvest demands while also allowing for longer-term forest regeneration.
At the University of Nairobi, successful urban forest islands with potential natural vegetation have been established using the “Miyawaki method” (Miyawaki, 2004). This method uses native trees to restore indigenous forests at timelines shorter than if natural regeneration was allowed to take its course (Figure 10.D). To create an urban green space on the university property, we selected 16 native tree species using a vegetation science study of remnant forests around Nairobi. Within 16 months, many of the trees had established well, with the best performing species, Ehretia cymosa, growing to over 2 m (Kiboi et. al., 2014). This study illustrates the importance of selecting locally adapted species in forest restoration initiatives.
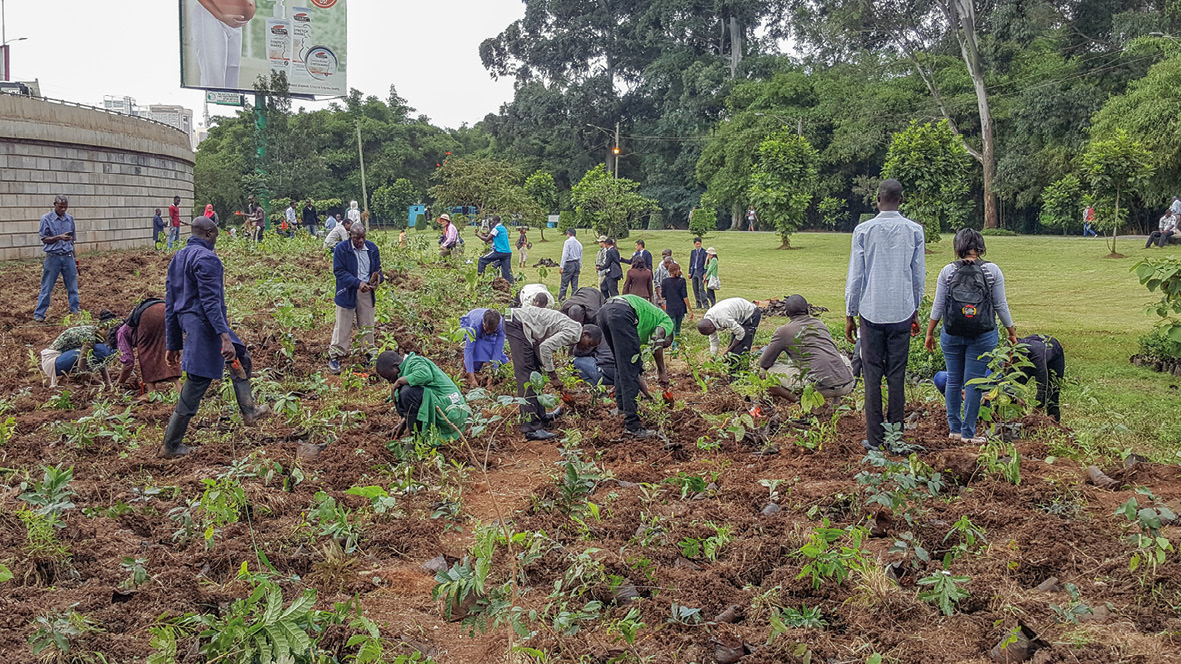
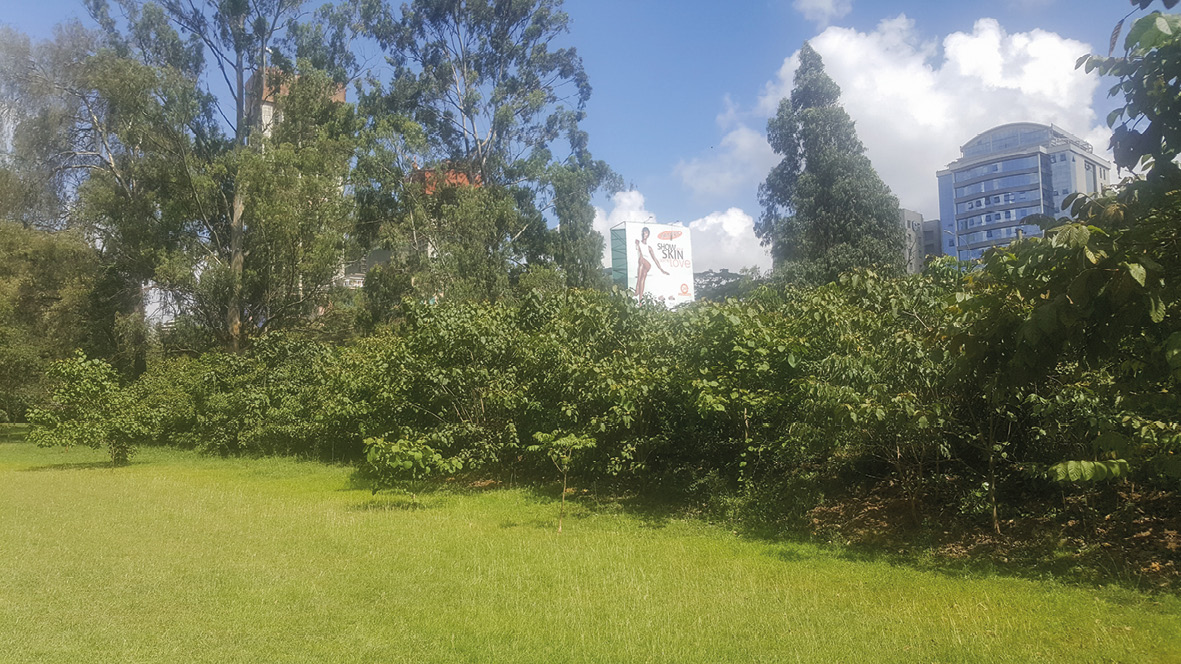
Figure 10.D (Top) June 2016: project participants planting trees on the University of Nairobi, Chiromo campus, following the Miyawaki method; (Bottom). January 2019: less than three years later, the trees have successfully grown to provide ecosystem services to the Chiromo campus. Photographs by Samuel Kiboi, CC BY 4.0.
Sustainable restoration practices can alleviate the short-term pressure from restored ecosystems while they mature to a self-sustaining structure. Not only should locally adapted species be promoted, but also native species that can be continuously coppiced, where new shoots rapidly replace harvested branches and portions of branches. Exotic species, such as Australian gum, pine, and mesquite (Prosopis juliflora) often display these characteristics, but those species are often invasive with detrimental effects on native ecosystems and communities. Fortunately, many African plant species are also good candidates for sustainable restoration initiatives, including camphor bush (Tarchonanthus camphoratus), sickle-leaved false-thorn (Albizia harveyi), silver clusterleaf (Terminalia sericea), and weeping wattle (Peltophorum africanum) (Kennedy, 1998; Kaschula et al., 2005). Although coppices may have more variable increases in biomass compared to initial planted stands, it is a sustainable way of biomass management especially in areas that experience high demand for harvestable wood. In addition, planting native trees and shrubs in farmlands typically provides beneficial ecosystem services through increasing the abundance and diversity of native insectivorous birds and pollinators of crops. In these various ways, the right management practices can lead to benefits for local people, biodiversity, and sustainable conservation practices.
Tropical forests: Tropical forests cover less than 10% of Earth’s land surface; yet, they contain more than half of all terrestrial species (Cortlett and Primack, 2011). When these forests are lost, we lose substantial biodiversity and ecosystem services. For this reason, tropical forest restoration initiatives in Africa and elsewhere have received much attention in recent years. Towards the end of this chapter we will discuss a major global effort focussed on restoring degraded tropical forests, known as REDD+.
Africa has already lost over 40% of its wetlands through human activity, with current loss rates among the highest in the world.
Wetlands: Africa has already lost over 40% of its wetlands through human activity, with current loss rates among the highest in the world (Davidson, 2014). Because of the recognised importance of wetlands in providing flood control and other ecosystem services (Section 5.5.3), damaged wetlands are frequently targeted in restoration efforts. Wetlands are defined by their hydrology; therefore, wetland restoration projects often the focus on restoring a site’s original hydrology. One such example comes from South Africa, where authorities (with support from the World Bank) have been working on restoring Africa’s largest estuarine lake at iSimangaliso Wetland Park—a multistep process that involves restoration of the estuary’s hydrology, controlling invasive plants around the wetland, and improving farming practices in the surrounding area (Whitfield et al., 2013). Wetland restoration can also occur through activities like dam removals (Section 11.3.2) or replacing exotic vegetation that deplete groundwater with native vegetation to promote groundwater retention (Sirami et al., 2013). Importantly, true wetland restorations are notoriously difficult to accomplish. It can be relatively easy to replant a wetland to look as it previously looked, but to restore the foundational hydrology often requires sophisticated engineering. In many cases, partial wetland rehabilitation is the best that can be achieved.
Mangrove swamps (Figure 10.11) provide nursery grounds for many economically important fisheries, protect coastal communities against powerful storms, and prevent saltwater from intruding into freshwater systems (van Bochove et al., 2014). They are also among the world’s most important carbon sinks, storing four times more carbon per hectare than other types of tropical forests (Donato et al., 2011). Yet, over 35% of the world’s mangrove swamps have already been degraded by agriculture, urban expansion, pollution, and commercial shellfish farming (MEA, 2005; Giri et al., 2011). To regain these lost services, several communities are now restoring their mangroves, while also adopting more sustainable practices to reduce damage to these important habitats (Feka et al., 2009). One of Africa’s most ambitious mangrove restoration projects have been initiated in Senegal, where more than 300,000 local citizens planted more than 150 million mangrove trees across 140 km2 between 2006 and 2013 (Cormier-Salem and Panfili, 2016). Mangrove (as any other) restoration projects do need to be planned carefully to ensure success. For example, it is important to choose ecologically-appropriate species to plant, rather than the fastest growing species that promises quick (but not necessarily optimal) results. Studies from Eritrea have also shown how fertiliser runoff caused by wave action could reduce lead to project failure (Sato et al., 2005). Another concern is that mangroves are often exploited, restored, and managed as forests, while the primary determinants of their function and structure—hydrology, soils, and nutrients—are neglected (Lewis, 2005; Gopal, 2013). Recent work showed that natural regeneration of mangrove swamps may produce more diverse, resilient, and productive ecosystems compared to planting efforts (Wetlands International, 2016). These issues will need to be addressed to ensure the long-term sustainability of mangrove restoration efforts.
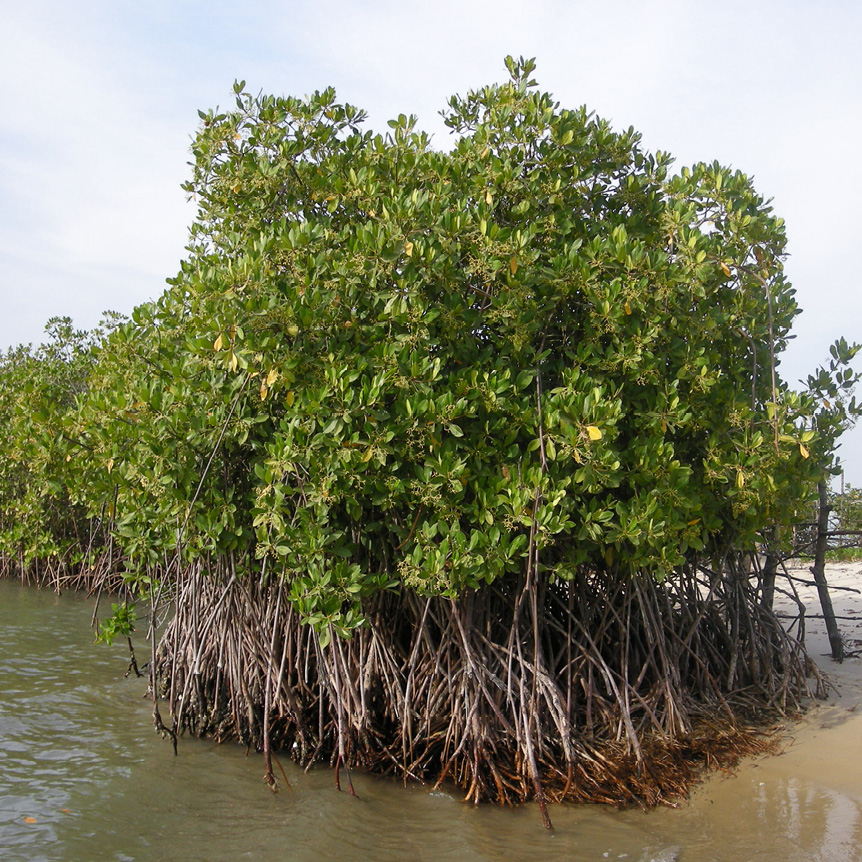
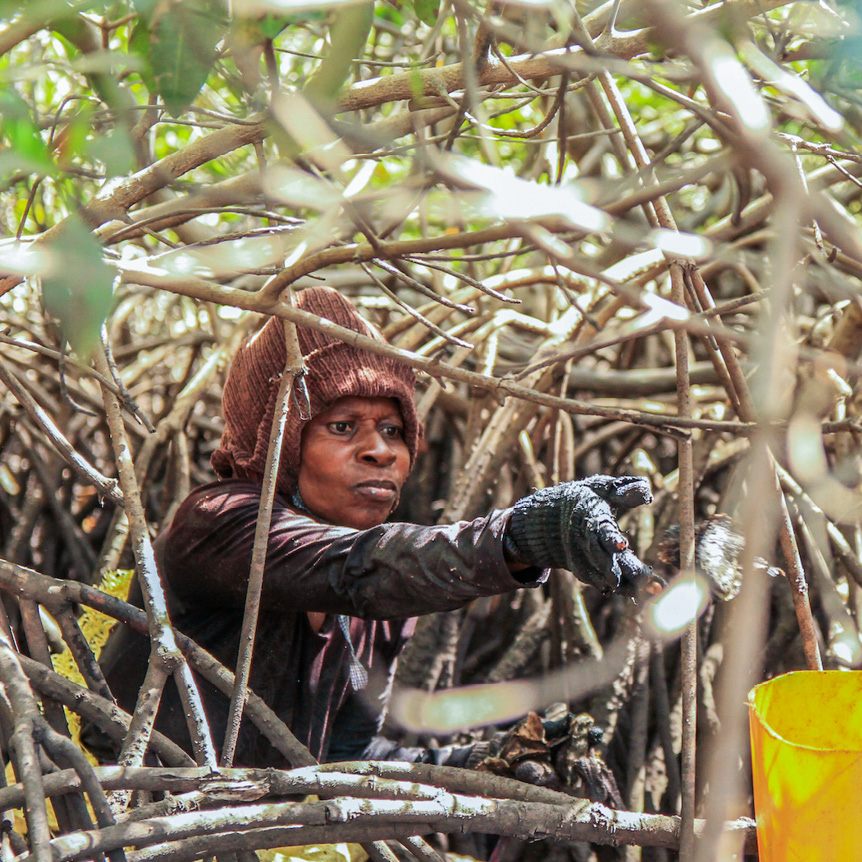
Figure 10.11 (Left) Classic air-breathing roots of a mangrove tree in Senegal. Photograph by Ji-Elle, https://commons.wikimedia.org/wiki/File:Carabane-Mangrove.JPG, CC0. (Right) A woman collecting oysters among mangroves in Senegal’s Saloum Delta. Photograph by Julien Saison, https://commons.wikimedia.org/wiki/File:Cueilleuse_traditionnelle_d%27Hu%C3%AEtres_de_mangrove,_Sine_Saloum,_femme_du_village_de_Soucouta,_S%C3%A9n%C3%A9gal.jpg, CC by-SA 4.0.
Seasonal drylands: Through extensive land mismanagement (primarily overgrazing and unsustainable agriculture), a large portion of Africa’s seasonal drylands are undergoing desertification, the conversion of once-productive land to desolate man-made deserts—large dry unproductive dust bowls with no vegetation. The degradation of these lands has crippled agriculture, obliterated natural biological communities, and displaced millions of people. While many drylands seem to regenerate naturally when pressures associated with land mismanagement are removed at an early stage, extended periods of mismanagement hamper recovery by leading to a loss of natural seed banks, nutrients, and microsites that allow for seedling establishment.
Somalia is home to one of the world’s most effective desertification reversal programmes. Since the early 1990s, when Somalia’s national government collapsed, Somalis have been tormented by warlords and civil war. The lack of effective governance also saw the rise of an unregulated charcoal trade; groves of thorn trees hundreds of years old were set ablaze, before the so-called “black gold” was exported to Arabia. The resultant wildfires and removal of trees caused an erosion crisis, turning grazing lands that once supported a diverse pastoralist community into unproductive wastelands. The resulting famine, exacerbated by droughts, caused even more Somalis to turn to a life of crime, piracy, and terrorism in a desperate effort to support their families. To reverse this decline, the humanitarian NGO Adeso successfully persuaded a regional government to create and enforce a ban on charcoal exports. Adeso also started educating local people about the links between the environment and their own lives, and introduced sustainable alternatives to the charcoal trade, such as promoting the use of solar cookers to reduce the need for charcoal fuel. To reverse desertification and prevent further erosion, Adeso showed local communities how to construct small and simple rock dams; the dams also provide a microenvironment suitable for thorn tree seeds to germinate. Adeso has been so successful in these ventures that they subsequently expanded their work to Kenya and South Sudan.
Coral Reefs: Coral reefs are one of the world’s most important marine ecosystems, both ecologically and economically. They provide food to local communities, support ecotourism industries, and protect coasts by reducing wave energy by as much as 97% (Ferrario et al., 2014). Yet, coral reefs are also one of the most threatened marine ecosystems, impacted heavily by overharvesting, pollution, sedimentation, and climate change. Nevertheless, restoring coral reefs is well worth it; a meta-analysis found that it is nearly 20 times cheaper to restore coral reefs than to construct artificial systems for coastal protection (Ferrario et al., 2014). As such, several initiatives are now in progress to restore coral reefs, ranging from transplanting corals and boosting sea urchin populations for seaweed control to creating artificial reefs that can act as substrate for coral settlements (Lindahl, 2003; Edwards and Gomez, 2007).
10.3.3 The future of ecological restoration
Research in restoration ecology has grown rapidly in recent years. Many reviews (e.g. Suding, 2011) and books (e.g. Falk et al., 2016) have recently been published on the topic. The Society for Ecological Restoration (SER) was established in 1988 to support the field, and two scientific journals (Restoration Ecology and Ecological Restoration) publish hundreds of papers each year on the topic, in addition to the papers published in other ecological and conservation journals. The growth in research provides scientists and land managers more studies and evidence to inform planning and improvement of restoration projects.
A recent development in the field involves biodiversity offsets (ten Kate et al., 2004; MacFarlane et al., 2016). A system generally used by developers, biodiversity offsets aim to achieve no net loss of biodiversity during economic development; some projects even aim for a net overall biodiversity gain. Developers accomplish this by compensating for the ecosystem damage (or loss of threatened species populations, Kormos et al., [2014]) that may be incurred during a development project. This compensation usually follows one or more of three main strategies: (1) reducing the extent of damage at the development site, (2) restoring or protecting natural communities at a different “receptor site” as compensation for what is being lost, and (3) enhancement of the remaining natural communities after development.
While biodiversity offsets (and other restoration initiatives in general) sound good in theory, it is important to remember that the most effective biodiversity conservation strategy remains protecting and managing intact ecosystems. Studies and practical experience have shown that ecological restoration efforts often fail to recreate key characteristics of their reference sites, including species composition or ecosystem functioning, even after years of effort and investment. It is also important to remember than some African ecosystems regenerate very slowly—tropical forests require more than 100 years to develop (Bonnell et al., 2011)—so even effective restorations may take decades to provide the full range of benefits. In cases where biodiversity offsets are pursued, it is critical to ensure that these initiatives indeed offer true conservation gains by mitigating the various associated risks (Coralie et al., 2015; Gordon et al., 2015; Maron et al., 2016).
Because restoring damaged environments takes considerable time and resources, preserving intact ecosystems should be prioritised.
10.4 Combatting Climate Change Through Ecosystem Conservation
Complex natural ecosystems play an important role in mitigating the destructive effects of climate change. Prominently, living plants sequester greenhouse gases from the atmosphere (Zarin et al., 2015); in contrast, their loss due to habitat loss increases greenhouse gas emissions (Section 6.1). Studies have also shown how ecosystems with high complexity (Betts et al. 2018) and species diversity (Mokany et al., 2014; Isbell et al., 2015) are better buffered against climate change. Lastly, by maintaining and restoring carbon-sequestering ecosystems, we also provide opportunities for climate-sensitive to persist despite the threat of climate change pace (Section 11.4).
One of the foremost initiatives aimed at combatting climate change through ecosystem conservation and restoration is known as the Reducing Emissions from Deforestation and Forest Degradation (REDD+, http://www.un-redd.org) programme. Set up by the UN, REDD+ provides financial incentives to local communities and landowners that make conservation of carbon-sequestering ecosystems worth more than destroying them. Funding for REDD+ is obtained through carbon trading programmes, in which individuals and organisations looking to offset their emissions buy carbon credits. The funds obtained through REDD+ are then invested in initiatives that promote ecological restoration and reduce local dependence of intact ecosystems by creating alternative income streams such as sustainable crop, timber, honey, milk, and meat production.
REDD+ provides financial incentives to local communities and landowners by making conservation of carbon-sequestering ecosystems worth more than destroying them.
The original aim of REDD+ was to safeguard primary old-growth forests, but the diversity of goals set out by REDD+ also include improving ecosystem connectivity, protecting threatened species, and preventing further loss and degradation of carbon-sequestering ecosystems other than forests. Africa has been a major beneficiary of this programme. Since its inception in 2007, REDD+ projects have been funded in 27 Sub-Saharan African countries (http://www.reddprojectsdatabase.org), affording protection for over 1.6 million ha of forest (Panfil and Harvey, 2016), and providing opportunities for thousands of Africans who would not have had access to these funds otherwise.
Yet the future of these opportunities is not secure. Concerns exist regarding the effectiveness of REDD+ programmes, much of which is based on problematic implementation, long-term funding security, lack of monitoring, and lack of concrete conservation goals (Phelps et al., 2011; Panfil and Harvey. 2015; Fletcher et al., 2016). For example, there are concerns that REDD+ programmes can develop into a form of perverse subsidies, such as when native vegetation is cleared to establish plantations (Figure 10.12) with trees that have a high risk of becoming invasive (Lindenmayer et al., 2012). Similarly, there are concerns about the strong emphasis on forests, possibly at the expense of other important ecosystems and ecosystem services (Bond, 2016). Conservation biologists continue to be hopeful that REDD+, as with the range of approaches described in this chapter, will provide opportunities for land managers and scientists to successfully protect and restore biodiversity now and into the future.
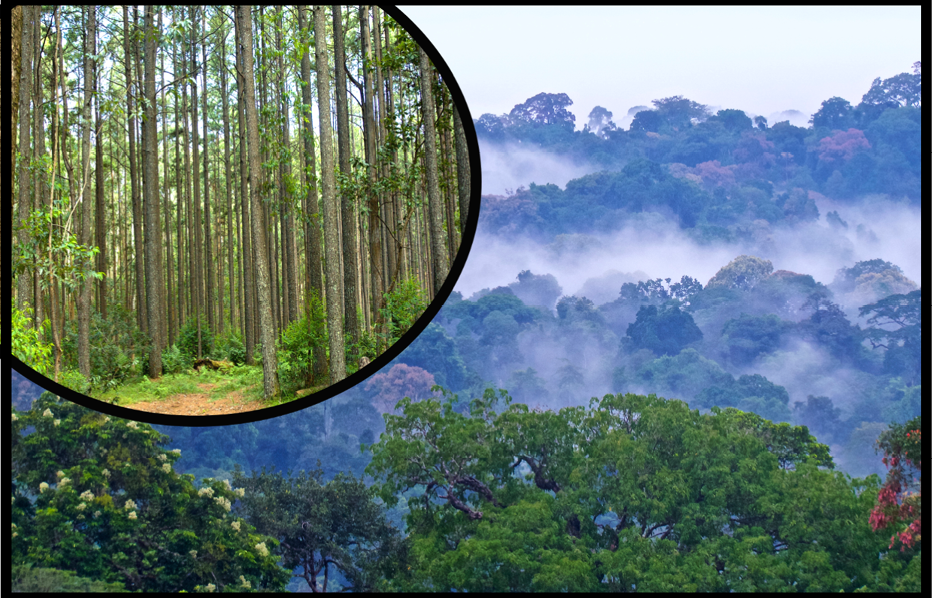
Figure 10.12 The Reducing Emissions from Deforestation and Forest Degradation (REDD+) programme aims to promote forest conservation by paying landowners to protect forests on their lands. However, there are concerns that some REDD+ funds have become a form of perverse subsidies, for example when native vegetation is cleared to establish plantations of invasive trees. To ensure programme sustainability, it is important to strike a balance between meeting short-term development goals and ensuring the protection of a complex and adaptable biological landscape that can provide a variety of ecosystem services over the long term. Photographs by Johnny Wilson, CC BY 4.0.
10.5 Summary
- Ecosystem conservation and management involves three different activities: (1) monitoring ecosystem components, (2) maintaining healthy ecosystems, and (3) restoring damaged ecosystems.
- An ecosystem in which all the chemical, physical, and biological components and processes are functioning normally is considered healthy. Ecosystems that remain healthy through disturbance are resistant, while ecosystems that rapidly recover after disturbance are resilient.
- Ecosystems can be monitored using direct observation, environmental or biochemical indicators, and remote sensing analysis. It is important that any monitoring method be consistent and repeatable across space and time.
- To maintain ecosystems that can support diverse ecological communities, conservationists are guided by three complementary management principles: (1) maintain critical ecosystem processes (water cycling, nutrient cycling, energy flow, community dynamics), (2) minimise external threats, and (3) be adaptive yet minimally intrusive.
- Ecological restoration is the practice of restoring damaged ecosystems to an agreed-upon benchmark. This can be accomplished via rehabilitation, partial restoration, complete restoration, or taking no action. The strategy followed will depend on each project’s goals and resource availability.
10.6 Topics for Discussion
- Read the articles by Bunce et al. (2008) and/or Papworth et al. (2009) about shifting baselines. Then think of a natural ecosystem in your region; it could even be an ecosystem in a protected area. How do you think that ecosystem looked 50 years ago? What about 100 years ago? And 1,000 years ago? At what time do you think the ecosystem was able to support the most diverse ecological community? What would you do to restore (or maintain) the ecosystem to this state? Would such a restoration project impact some species negatively? Is that a problem?
- Consider all the aquatic communities in your region (ponds, marshes, streams, rivers, lakes, estuaries, coastal waters, etc.). Who is responsible for managing these ecosystems, and how do they balance the need for protecting biodiversity with the needs of society for natural resources? What additional conservation projects would you implement to help protect those ecosystems in the coming decades?
- Imagine that the last population of a threatened bird species (which draws birdwatchers to the area) lives along a river nearby. This river also has numerous endemic species of fish, shellfish, and insects. A foreign company recently obtained permission to dam the river for hydropower generation. Beyond the impact of flooding, the dam will also cause various forms of pollution which will destroy the threatened birds’ food source and nesting area. Upon writing about the challenge in the local newspaper, you receive US $1 million from an anonymous donor to save the bird. The company is willing to forego the development in exchange for the US $1 million. It will cost an additional $750,000 to implement an effective ecological restoration programme that can reverse the threatened birds’ population declines. Is it better to buy out the company and not devote additional resources to ecological restoration and researching the bird? Or would you rather spend the money on finding alternative ways to protect the bird and the other endemic species? Explain your answers.
10.7 Suggested Readings
Crouzeilles, R., M.S. Ferreira, R.L. Chazdon, et al. 2017. Ecological restoration success is higher for natural regeneration than for active restoration in tropical forests. Science Advances 3: e1701345. https://doi.org/10.1126/sciadv.1701345 Natural regeneration is an appropriate restoration strategy under the right conditions.
Maron, M., C.D. Ives, H. Kujala, et al. 2016. Taming a wicked problem: Resolving controversies in biodiversity offsetting. BioScience 66: 489–98. https://doi.org/10.1093/biosci/biw038 Biodiversity offsets offer conservation opportunities as well as challenges.
Miller, B.P., E.A. Sinclair, M.H.M. Menz, et al. 2017. A framework for the practical science necessary to restore sustainable, resilient, and biodiverse ecosystems. Restoration Ecology 25: 605–17. https://doi.org/10.1111/rec.12475 Practical guidelines for ecological restoration success.
Panfil, S.N., and C.A. Harvey. 2015. REDD+ and biodiversity conservation: A review of the biodiversity goals, monitoring methods, and impacts of 80 REDD+ projects. Conservation Letters 9: 143–50. https://doi.org/10.1111/conl.12188 The UN’s REDD+ programme shows promise, but several shortcomings need to be addressed.
van Wilgen, B.W., and A. Wannenburgh. 2016. Co-facilitating invasive species control, water conservation and poverty relief: Achievements and challenges in South Africa’s Working for Water programme. Current Opinion in Environmental Sustainability 19: 7–17. https://doi.org/10.1016/j.cosust.2015.08.012 Ecosystem conservation, poverty relief, and job creation.
van Wilgen, B.W., and D.M. Richardson. 2014. Challenges and trade-offs in the management of invasive alien trees. Biological Invasions 16: 721–34. https://doi.org/10.1007/s10530-013-0615-8 Is the benefits gained from planting invasive species worth the costs?
Waldram, M.S., W.J. Bond, and W.D. Stock. 2008. Ecological engineering by a mega- grazer: White rhino impacts on a South African savanna. Ecosystems 11: 101–12. https://doi.org/10.1007/s10021-007-9109-9 Restoring ecosystem engineer populations results in a more diverse landscape.
Zachariades, C., I.D. Paterson, L.W. Strathie, et al. 2017. Assessing the status of biological control as a management tool for suppression of invasive alien plants in South Africa. Bothalia 47: 1–19. http://dx.doi.org/10.4102/abc.v47i2.2142 Biocontrol has many benefits
One of the following two manuscripts:
van Wilgen, B.W., and H.C. Biggs. 2011. A critical assessment of adaptive ecosystem management in a large savanna protected area in South Africa. Biological Conservation 144: 1179–87. https://doi.org/10.1016/j.biocon.2010.05.006 A general overview of an adaptive management programme.
van Wilgen, B.W., N. Govender, I.P.J. Smit, et al. 2014. The ongoing development of a pragmatic and adaptive fire management policy in a large African savanna protected area. Journal of Environmental Management 132: 358-368. http://dx.doi.org/10.1016/j.jenvman.2013.11.003 A specific overview of an adaptive management programme.
Bibliography
Acquah-Lamptey, D., R. Kyerematen, and E.O. Owusu. 2013. Using odonates as markers of the environmental health of water and its land related ecotone. International Journal of Biodiversity and Conservation 5: 761–69
Akello, J., T. Dubois, D. Coyne, et al. 2008. Endophytic Beauveria bassiana in banana (Musa spp.) reduces banana weevil (Cosmopolites sordidus) fitness and damage. Crop Protection 27: 1437–41. https://doi.org/10.1016/j.cropro.2008.07.003
Akutse, K.S., N.K. Maniania, K.K.M. Fiaboe, et al. 2013. Endophytic colonization of Vicia faba and Phaseolus vulgaris (Fabaceae) by fungal pathogens and their effects on the life-history parameters of Liriomyza huidobrensis (Diptera: Agromyzidae). Fungal Ecology 6: 293–301. https://doi.org/10.1016/j.funeco.2013.01.003
Allan, D.G., J.A. Harrison, R.A. Navarro, et al. 1997. The impact of commercial afforestation on bird populations in Mpumalanga Province, South Africa—Insights from bird atlas data. Biological Conservation 79:173–85. https://doi.org/10.1016/S0006-3207(96)00098-5
Archibald, S., R.J. Scholes, D.P. Roy, et al. 2010. Southern African fire regimes as revealed by remote sensing. International Journal of Wildland Fire 19: 861–78. https://doi.org/10.1071/WF10008
Bester, M.N., J.P. Bloomer, R.J. van Aarde, et al. 2002. A review of the successful eradication of feral cats from sub-Antarctic Marion Island, Southern Indian Ocean. South African Journal of Wildlife Research 32: 65–73. https://hdl.handle.net/10520/EJC117137
Betts, M.G., B. Phalan, S.J.K. Frey, et al. 2018. Old‐growth forests buffer climate‐sensitive bird populations from warming. Diversity and Distributions 24: 439–47. https://doi.org/10.1111/ddi.12688
Bhagwat S.A., E. Breman, T. Thekaekara, et al. 2012. A battle lost? Report on two centuries of invasion and management of Lantana camara L. in Australia, India and South Africa. PLoS ONE 7: e32407. https://doi.org/10.1371/journal.pone.0032407
Bodin, N., R. N’Gom-Kâ, S. Kâ, et al. 2013. Assessment of trace metal contamination in mangrove ecosystems from Senegal, West Africa. Chemosphere 90: 150–57. https://doi.org/10.1016/j.chemosphere.2012.06.019
Bond, W.J. 2016. Ancient grasslands at risk. Science 351: 120–22. https://doi.org/10.1126/science.aad5132
Bonnell, T.R., R. Reyna-Hurtado, and C.A. Chapman. 2011. Post-logging recovery time is longer than expected in an East African tropical forest. Forest Ecology and Management 261: 855–64. https://doi.org/10.1016/j.foreco.2010.12.016
Bornman, M.S., and H. Bouwman. 2012. Environmental pollutants and diseases of sexual development in humans and wildlife in South Africa: Harbingers of impact on overall health? Reproduction in Domestic Animals 47: 327–32. https://doi.org/10.1111/j.1439-0531.2012.02094.x
Bownes, A., M.P. Hill, and M.J. Byrne. 2013. The role of nutrients in the responses of water hyacinth, Eichhornia crassipes (Pontederiaceae) to herbivory by a grasshopper Cornops aquaticum Brüner (Orthoptera: Acrididae). Biological Control 67: 555–62. https://doi.org/10.1016/j.biocontrol.2013.07.022
Bradshaw, A.D. 1990. The reclamation of derelict land and the ecology of ecosystems. In: Restoration Ecology: A Synthetic Approach to Ecological Research, ed. by W.R. Jordan III et al. (Cambridge: Cambridge University Press).
Buckland, S.T., and A. Johnston. 2017. Monitoring the biodiversity of regions: Key principles and possible pitfalls. Biological Conservation 214: 23–34. https://doi.org/10.1016/j.biocon.2017.07.034
Bunce, M., L.D. Rodwell, R. Gibb, et al. 2008. Shifting baselines in fishers’ perceptions of island reef fishery degradation. Ocean and Coastal Management 51: 285–302. https://doi.org/10.1016/j.ocecoaman.2007.09.006
Burton, M.E.H., J.R. Poulsen, M.E. Lee, et al. 2017. Reducing carbon emissions from forest conversion to oil palm agriculture in Gabon, Conservation Letters 10: 297–307. https://doi.org/10.1111/conl.12265
Carreiras, J.M.B, M.J. Vasconcelos, and R.M. Lucas. 2012. Understanding the relationship between aboveground biomass and ALOS PALSAR data in the forests of Guinea-Bissau (West Africa). Remote Sensing of Environment 121: 426–42. https://doi.org/10.1016/j.rse.2012.02.012
Chislock, M.F., E. Doster, R.A. Zitomer, et al. 2013. Eutrophication: Causes, consequences, and controls in aquatic ecosystems. Nature Education Knowledge 4: 10.
Coetzee, J.A., A. Bownes, and G.D. Martin. 2011. Prospects for the biological control of submerged macrophytes in South Africa. African Entomology 19: 469–88. https://doi.org/10.4001/003.019.0203
Coetzee, J.A., and M.P. Hill. 2012. The role of eutrophication in the biological control of water hyacinth, Eichhornia crassipes, in South Africa. BioControl 57: 247–61. https://doi.org/10.1007/s10526-011-9426-y
Coralie, C., O. Guillaume, and N. Claude. 2015 Tracking the origins and development of biodiversity offsetting in academic research and its implications for conservation: A review. Biological Conservation 192: 492–503. https://doi.org/10.1016/j.biocon.2015.08.036
Corlett, R., and R.B. Primack. 2011. Tropical Rain Forests: An Ecological and Biogeographical Comparison (Hoboken: Wiley-Blackwell). https://doi.org/10.1002/9781444392296
Cormier-Salem, M.-C., and J. Panfili. 2016. Mangrove reforestation: Greening or grabbing coastal zones and deltas? Case studies in Senegal. African Journal of Aquatic Science 41: 89–98. https://doi.org/10.2989/16085914.2016.1146122
Crouzeilles, R., M.S. Ferreira, R.L. Chazdon, et al. 2017. Ecological restoration success is higher for natural regeneration than for active restoration in tropical forests. Science Advances 3: e1701345. https://doi.org/10.1126/sciadv.1701345
Cumming, D.H.M., and G.S. Cumming. 2015. One Health: An ecological and conservation perspective. In: One Health: The Theory and Practice of Integrated Health Approaches, ed. by J. Zinsstag, et al. (Wallingford: CAB International).
Davidson, N.C. 2014. How much wetland has the world lost? Long-term and recent trends in global wetland area. Marine and Freshwater Research 65: 934–41. https://doi.org/10.1071/MF14173
Davies, A.B., C.J. Tambling, G.I.H. Kerley, et al. 2016. Effects of vegetation structure on the location of lion kill sites in African thicket. PloS ONE 11: e0149098. https://doi.org/10.1371/journal.pone.0149098
de Groote, H., O. Ajuonu, S. Attignon, et al. 2003. Economic impact of biological control of water hyacinth in Southern Benin. Ecological Economics 45: 105–17. https://doi.org/10.1016/S0921-8009(03)00006-5
de Lange, W.J., and B.W. van Wilgen. 2010. An economic assessment of the contribution of biological control to the management of invasive alien plants and to the protection of ecosystem services in South Africa. Biological Invasions 12: 4113–24. https://doi.org/10.1007/s10530-010-9811-y
de Wit, M., H. van Zyl, D. Crookes, et al. 2009. Investing in natural assets: A business case for the environment in the City of Cape Town (Cape Town: City of Cape Town).
Di Marco, M., G.M. Buchanan, Z. Szantoi, et al. 2014. Drivers of extinction risk in African mammals: The interplay of distribution state, human pressure, conservation response and species biology. Philosophical Transactions of the Royal Society B 369: 20130198. https://doi.org/10.1098/rstb.2013.0198
Donato, D.C., J.B. Kauffman, D. Murdiyarso, et al. 2011. Mangroves among the most carbon-rich forests in the tropics. Nature Geoscience 4: 293–97. https://doi.org/10.1038/ngeo1123
Drechsel, P., L. Gyiele, D. Kunze, et al. 2001. Population density, soil nutrient depletion, and economic growth in sub-Saharan Africa. Ecological Economics 38: 251–58. https://doi.org/10.1016/S0921-8009(01)00167-7
Dube, T., O. Mutanga, K. Seutloali, et al. 2015. Water quality monitoring in sub-Saharan African lakes: A review of remote sensing applications. African Journal of Aquatic Science 40: 1–7. https://doi.org/10.2989/16085914.2015.1014994
Dubovyk, O., T. Landmann, B.F.N. Erasmus, et al. 2015. Monitoring vegetation dynamics with medium resolution MODIS-EVI time series at sub-regional scale in southern Africa. International Journal of Applied Earth Observation and Geoinformation 38: 175–83. https://doi.org/10.1016/j.jag.2015.01.002
Edwards, A.J., and E.D. Gomez. 2007. Reef Restoration Concepts and Guidelines: Making Sensible Management Choices in the Face of Uncertainty (St. Lucia: Coral Reef Targeted Research and Capacity Building for Management Programme).
Estes, R.D., J.L. Atwood, and A.B. Estes. 2006. Downward trends in Ngorongoro Crater ungulate populations 1986–2005: Conservation concerns and the need for ecological research. Biological Conservation 131: 106–20. https://doi.org/10.1016/j.biocon.2006.02.009
Falk, D.A., M.A. Palmer, and J.B. Zedler. 2016. Foundations of Restoration Ecology (Washington: Island Press).
Feka, N.Z., G.B. Chuyong, and G.N. Ajonina. 2009. Sustainable utilization of mangroves using improved fish-smoking systems: A management perspective from the Douala-Edea wildlife reserve, Cameroon. Tropical Conservation Science 2: 450–68. https://doi.org/10.1177/194008290900200406
Ferrario, F., M.W. Beck, C.D. Storlazzi, et al. 2014. The effectiveness of coral reefs for coastal hazard risk reduction and adaptation. Nature Communications 5: 3794. https://doi.org/10.1038/ncomms4794
Fletcher, R., W. Dressler, B. Büscher, et al. 2016. Questioning REDD+ and the future of market‐based conservation. Conservation Biology 30: 573–675. https://doi.org/10.1111/cobi.12680
Furukawa, T., K. Fujiwara, S. Kiboi, et al. 2011. Can stumps tell what people want: Pattern and preference of informal wood extraction in an urban forest of Nairobi, Kenya. Biological Conservation 144: 3047–54. https://doi.org/10.1016/j.biocon.2011.09.011
Galatowitsch, S., and D.M. Richardson. 2005. Riparian scrub recovery after clearing of invasive alien trees in headwater streams of the Western Cape, South Africa. Biological Conservation 122: 509–21. https://doi.org/10.1016/j.biocon.2004.09.008
Garcia-Carreras, L., and D.J. Parker. 2011. How does local tropical deforestation affect rainfall? Geophysical Research Letters 38: L19802. https://doi.org/10.1029/2011GL049099
Garcin, Y., P. Deschamps, G. Ménot, et al. 2018. Early anthropogenic impact on West Central African rainforests 2,600 years ago. Proceedings of the National Academy of Sciences 115: 3261–66. https://doi.org/10.1073/pnas.1715336115
Gerlach, J. 2014. Prodida stella. The IUCN Red List of Threatened Species 2014: e.T196234A2443094. http://doi.org/10.2305/IUCN.UK.2014-1.RLTS.T196234A2443094.en
Giri, C., E. Ochieng, L.L. Tieszen, et al. 2011. Status and distribution of mangrove forests of the world using Earth observation satellite data. Global Ecology and Biogeography 20: 154–59. https://doi.org/10.1111/j.1466-8238.2010.00584.x
Goldammer, J.G., and C. de Ronde. 2004. Wildland Fire Management Handbook for Sub-Sahara Africa (Freiburg: GFMC). http://gfmc.online/latestnews/GFMC-Wildland-Fire-Management-Handbook-Sub-Sahara-Africa-2004.pdf
Gopal, B. 2013. Mangroves are wetlands, not forests: Some implications for their management. In: Mangrove Ecosystems of Asia, ed. by I. Faridah-Hanum, et al. (New York: Springer). https://doi.org/10.1007/978-1-4614-8582-7
Gordon, A., J.W. Bull, C. Wilcox, et al. 2015. Perverse incentives risk undermining biodiversity offset policies. Journal of Applied Ecology 52: 532–37. https://doi.org/10.1111/1365-2664.12398
Güereña, D., H. Neufeldt, J. Berazneva, et al. 2015. Water hyacinth control in Lake Victoria: Transforming an ecological catastrophe into economic, social, and environmental benefits. Sustainable Production and Consumption 3: 59–69. https://doi.org/10.1016/j.spc.2015.06.003
Diop, O., and M.P. Hill, M.P. 2009. Quantitative post-release evaluation of biological control of floating fern, Salvinia molesta DS Mitchell (Salviniaceae), with Cyrtobagous salviniae Calder and Sands (Coleoptera: Curculionidae) on the Senegal River and Senegal River Delta. African Entomology 17: 64–70. https://doi.org/10.4001/003.017.0108
Hopcraft, J.G.C., J.M. Morales, H.L. Beyer, et al. 2014. Competition, predation, and migration: Individual choice patterns of Serengeti migrants captured by hierarchical models. Ecological Monographs, 84: 355–72. https://doi.org/10.1890/13-1446.1
Isbell, F., D. Craven, J. Connolly, et al. 2015. Biodiversity increases the resistance of ecosystem productivity to climate extremes. Nature 526: 574–77. https://doi.org/10.1038/nature15374
IUCN. 2019. The IUCN Red List of Threatened Species. http://www.iucnredlist.org
Jeffery, K.J., L. Korte, F. Palla, et al. 2014. Fire management in a changing landscape: A case study from Lopé National Park, Gabon. Parks 20: 39–52. https://doi.org/10.2305/IUCN.CH.2014.PARKS-20-1.KJJ.en
Jenkins, A.R. 2005. Lesser Kestrel Falco naumanni. In: Roberts Birds of Southern Africa, ed. by P.A.R. Hockey, et al. (Cape Town: Trustees of the John Voelcker Bird Book Fund).
Kaschula, S.A., W.E. Twine, and M.C. Scholes. 2005. Coppice harvesting of fuelwood species on a South African common: Utilizing scientific and indigenous knowledge in community based natural resource management. Human Ecology 33: 387–418. https://doi.org/10.1007/s10745-005-4144-7
Kelly, L.T., and L. Brotons. 2017. Using fire to promote biodiversity. Science 355: 1264–65. https://doi.org/10.1126/science.aam7672
Kennedy, A.D. 1998. Coppicing of Tarconanthus camphoratus (Compositae) as a source of sustainable fuelwood production: An example from the Laikipia Plateau, Kenya. African Journal of Ecology 36: 148–58. https://doi.org/10.1046/j.1365-2028.1998.00115.x
Kiboi, S., K. Fujiwara, and P. Mutiso. 2014. Sustainable management of urban green environments: Challenges and opportunities. In: Sustainable Living with Environmental Risks, ed. by N. Kaneko, et al. (Tokyo: Springer). https://doi.org/10.1007/978-4-431-54804-1
Kohler, S., M. Connan, J. Hill, et al. 2011. Geographic variation in the trophic ecology of an avian rocky shore predator, the African Black Oystercatcher, along the southern African coastline. Marine Ecology Progress Series 435: 235–49. https://doi.org/10.3354/meps09215
Kormos, R., C.F. Kormos, T. Humle, et al. 2014. Great apes and biodiversity offset projects in Africa: The case for national offset strategies. PloS ONE 9: e111671. https://doi.org/10.1371/journal.pone.0111671
Kraaij, T., R.M. Cowling, B.W. Wilgen, et al. 2013. Proteaceae juvenile periods and post-fire recruitment as indicators of minimum fire return interval in eastern coastal fynbos. Applied Vegetation Science 16: 84–94. https://doi.org/10.1111/j.1654-109X.2012.01209.x
Kyerematen, R., D. Acquah-Lamptey, E.H. Owusu, et al. 2014. Insect diversity of the Muni-Pomadze Ramsar site: An important site for biodiversity conservation in Ghana. Journal of Insects 2014: 985684. http://doi.org/10.1155/2014/985684
Kyerematen, R., S. Adu-Acheampong, D. Acquah-Lamptey, et al. 2018. Butterfly diversity: An indicator for environmental health within the Tarkwa Gold mine, Ghana. Environment and Natural Resources Research 8: 69–83. https://doi.org/10.5539/enrr.v8n3p69
Laporte, N.T., J.A. Stabach, R. Grosch, et al. 2007. Expansion of industrial logging in Central Africa. Science 316: 1451–1451. https://doi.org/10.1126/science.1141057
Lawrence, D., and K. Vandecar. 2015. Effects of tropical deforestation on climate and agriculture. Nature Climate Change 5: 27–36. https://doi.org/10.1038/nclimate2430
Lewis, R.R. 2005. Ecological engineering for successful management and restoration of mangrove forests. Ecological Engineering 24: 403–18. https://doi.org/10.1016/j.ecoleng.2004.10.003
Lindahl, U. 2003. Coral reef rehabilitation through transplantation of staghorn corals: Effects of artificial stabilization and mechanical damages. Coral Reefs 22: 217–23. https://doi.org/10.1007/s00338-003-0305-6
Lindenmayer, D.B., K.B. Hulvey, R.J. Hobbs, et al. 2012. Avoiding bio‐perversity from carbon sequestration solutions. Conservation Letters 5: 28–36. https://doi.org/10.1111/j.1755-263X.2011.00213.x
Loarie, S.R., C.J. Tambling, and G.P. Asner. 2013. Lion hunting behaviour and vegetation structure in an African savanna. Animal Behaviour 85: 899–906. https://doi.org/10.1016/j.anbehav.2013.01.018
Lyons, A.J., W.C. Turner, and W.M. Getz. 2013. Home range plus: A space-time characterization of movement over real landscapes. Movement Ecology 1: 2. https://doi.org/10.1186/2051-3933-1-2
MacFarlane, D.M., S.D. Holness, A. von Hase, et al. 2016. Wetland offsets: A best-practice guideline for South Africa (Pretoria: SANBI and Department of Water and Sanitation). http://biodiversityadvisor.sanbi.org/wp-content/uploads/2014/09/Wetland-Offset-Guidelines-Version-7-For-stakeholder-comment.pdf
Maron, M., C.D. Ives, H. Kujala, et al. 2016. Taming a wicked problem: Resolving controversies in biodiversity offsetting. BioScience 66: 489–98. https://doi.org/10.1093/biosci/biw038
Martin, G.D., J.A. Coetzee, P.S.R. Weyl, et al. 2018. Biological control of Salvinia molesta in South Africa revisited. Biological Control 125: 7480. https://doi.org/10.1016/j.biocontrol.2018.06.011
Mashimbye, Z.E., M.A. Cho, J.P. Nel, et al. 2012. Model-based integrated methods for quantitative estimation of soil salinity from hyperspectral remote sensing data: A case study of selected South African soils. Pedosphere 22: 640–49. https://doi.org/10.1016/S1002-0160(12)60049-6
Masocha, M., A.K. Skidmore, X. Poshiwa, et al. 2011. Frequent burning promotes invasions of alien plants into a mesic African savanna. Biological Invasions 13: 1641–48. https://doi.org/10.1007/s10530-010-9921-6
Mbati, G., and P. Neuenschwander. 2005. Biological control of three floating water weeds, Eichhornia crassipes, Pistia stratiotes, and Salvinia molesta in the Republic of Congo.”BioControl 50: 635–45. https://doi.org/10.1007/s10526-004-5863-1
McClanahan, T., J.M. Maina, and N.A. Muthiga. 2011. Associations between climate stress and coral reef diversity in the western Indian Ocean. Global Change Biology 17: 2023–2032. https://doi.org/10.1111/j.1365-2486.2011.02395.x
MEA (Millenium Ecosystem Assessment). 2005. Ecosystems and Human Well-Being (Covello: Island Press). https://www.millenniumassessment.org
Miyawaki, A. 2004. Restoration of the living environment based on vegetation ecology: Theory and practice. Ecological Research 19: 83–90. https://doi.org/10.1111/j.1440-1703.2003.00606.x
Mokany, K., S. Prasad, and D.A. Westcott. 2014. Loss of frugivore seed dispersal services under climate change. Nature Communications 5: 4971. https://doi.org/10.1038/ncomms4971
Mwangi, E., and B. Swallow. 2008. Prosopis juliflora invasion and rural livelihoods in the Lake Baringo area of Kenya. Conservation and Society 6: 130–40. https://doi.org/10.4103/0972-4923.49207
Naidoo, L., M.A. Cho, R. Mathieu, et al. 2012. Classification of savanna tree species, in the Greater Kruger National Park region, by integrating hyperspectral and LiDAR data in a Random Forest data mining environment. ISPRS Journal of Photogrammetry and Remote Sensing 69: 167–79. https://doi.org/10.1016/j.isprsjprs.2012.03.005
NASA. 2009. ASTER Global Digital Elevation Model v. 2 (GDEM V2) (Pasadena: NASA JPL). https://doi.org/10.5067/ASTER/ASTGTM.002
NASA. 2013. NASA Shuttle Radar Topography Mission Global 3 Arc Second Sub-Sampled (SRTM3) (Sioux Falls: NASA LP DAAC). https://doi.org/10.5067/MEASURES/SRTM/SRTMGL3S.003
Nnoli, H., R. Kyerematen, S. Adu-Acheampong, et al. 2019. Change in aquatic insect abundance: Evidence of climate and land-use change within the Pawmpawm River in southern Ghana. Cogent Environmental Science 5: 1594511. https://doi.org/10.1080/23311843.2019.1594511
Panfil, S.N., and C.A. Harvey. 2015. REDD+ and biodiversity conservation: A review of the biodiversity goals, monitoring methods, and impacts of 80 REDD+ projects. Conservation Letters 9: 143–50. https://doi.org/10.1111/conl.12188
Papworth, S.K., J. Rist, L. Coad, et al. 2009. Evidence for shifting baseline syndrome in conservation. Conservation Letters 2: 93–100. https://doi.org/10.1111/j.1755-263X.2009.00049.x
Phelps, J., E.L. Webb, and L.P. Koh. 2011. Risky business: an uncertain future for biodiversity conservation finance through REDD+. Conservation Letters 4: 88–94. https://doi.org/10.1111/j.1755-263X.2010.00155.x
Pricope, N.G., and M.W. Binford. 2012. A spatio-temporal analysis of fire recurrence and extent for semi-arid savanna ecosystems in southern Africa using moderate-resolution satellite imagery. Journal of Environmental Management 100: 72–85. https://doi.org/10.1016/j.jenvman.2012.01.024
Rahlao, S.J., S.J. Milton, K.J. Esler, et al. 2010. The distribution of invasive Pennisetum setaceum along roadsides in western South Africa: The role of corridor interchanges. Weed Research 50: 537–43. https://doi.org/10.1111/j.1365-3180.2010.00801.x
Sanchez, P.A. 2010. Tripling crop yields in tropical Africa. Nature Geoscience 3: 299–300. https://doi.org/10.1038/ngeo853
Sato, G., A. Fisseha, S. Gebrekiros, et al. 2005. A novel approach to growing mangroves on the coastal mud flats of Eritrea with the potential for relieving regional poverty and hunger. Wetlands 25: 776. https://doi.org/10.1672/0277-5212(2005)025[0776:ANATGM]2.0.CO;2
Scantlebury, D.M., M.G.L. Mills, R.P. Wilson, et al. 2014. Flexible energetics of cheetah hunting strategies provide resistance against kleptoparasitism. Science 346: 79–81. https://doi.org/10.1126/science.1256424
Scott, J.M., B. Csuti, and F. Davis. 1991. Gap analysis: An application of Geographic Information Systems for wildlife species. In: Challenges in the Conservation of Biological Resources: A Practitioner’s Guide, ed. by D.J. Decker, et al. (Boulder: Westview Press).
Sirami, C., S.S. Jacobs, and G.S. Cumming. 2013. Artificial wetlands and surrounding habitats provide important foraging habitat for bats in agricultural landscapes in the Western Cape, South Africa. Biological Conservation 164: 30–38. https://doi.org/10.1016/j.biocon.2013.04.017
Smit, I.P.J., and H.H.T. Prins. 2015. Predicting the effects of woody encroachment on mammal communities, Grazing biomass and fire frequency in African savannas. PloS ONE 10: e0137857. https://doi.org/10.1371/journal.pone.0137857
Smit, I.P.J., C.C. Grant, and B.J. Devereux. 2007. Do artificial waterholes influence the way herbivores use the landscape? Herbivore distribution patterns around rivers and artificial water sources in a large African savanna park. Biological Conservation 136: 85–99. https://doi.org/10.1016/j.biocon.2006.11.009
Suding, K.N. 2011. Toward an era of restoration in ecology: Successes, failures and opportunities ahead. Annual Reviews in Ecology, Evolution, and Systematics 42: 465–87. https://doi.org/10.1146/annurev-ecolsys-102710-145115
Symeonakis, E., and N. Drake. 2004. Monitoring desertification and land degradation over sub-Saharan Africa. International Journal of Remote Sensing 25: 573–92. https://doi.org/10.1080/0143116031000095998
ten Kate, K., J. Bishop, and R. Bayon. 2004. Biodiversity offsets: Views, experience, and the business case (Gland: IUCN; London: Insight Investment). https://www.iucn.org/sites/dev/files/import/downloads/bdoffsets.pdf
Torres, J., J.C. Brito, M.J. Vasconcelos, et al. 2010. Ensemble models of habitat suitability relate chimpanzee (Pan troglodytes) conservation to forest and landscape dynamics in Western Africa. Biological Conservation 143: 416–25. http://doi.org/10.1016/j.biocon.2009.11.007
Uys R.G., W.J. Bond, and T.M. Everson. 2004. The effect of different fire regimes on plant diversity in southern African grasslands. Biological Conservation 118: 489–99. https://doi.org/10.1016/j.biocon.2003.09.024
van Bochove, J., E. Sullivan, and T. Nakamura. 2014. The Importance of Mangroves to People: A Call to Action (Cambridge: UNEP). http://wedocs.unep.org/handle/20.500.11822/9300
van Wilgen, B.W., and A. Wannenburgh. 2016. Co-facilitating invasive species control, water conservation and poverty relief: Achievements and challenges in South Africa’s Working for Water programme. Current Opinion in Environmental Sustainability 19: 7–17. http://doi.org/10.1016/j.cosust.2015.08.012
van Wilgen, B.W., and H.C. Biggs. 2011. A critical assessment of adaptive ecosystem management in a large savanna protected area in South Africa. Biological Conservation 144: 1179–87. https://doi.org/10.1016/j.biocon.2010.05.006
van Wilgen, B.W., and D.M. Richardson. 2014. Challenges and trade-offs in the management of invasive alien trees. Biological Invasions 16: 721–34. https://doi.org/10.1007/s10530-013-0615-8
van Wilgen, B.W., N. Govender, I.P.J. Smit, et al. 2014. The ongoing development of a pragmatic and adaptive fire management policy in a large African savanna protected area. Journal of Environmental Management 132: 358–68. http://dx.doi.org/10.1016/j.jenvman.2013.11.003
van Wilgen, B.W., G. Forsyth, and P. Prins. 2012. The management of fire-adapted ecosystems in an urban setting: The case of Table Mountain National Park, South Africa. Ecology and Society 17: 8. http://doi.org/10.5751/ES-04526-170108
van Wilgen, B.W., G.G. Forsyth, H. de Klerk, et al. 2010. Fire management in Mediterranean-climate shrublands: A case study from the Cape fynbos, South Africa. Journal of Applied Ecology 47: 631–38. https://doi.org/10.1111/j.1365-2664.2010.01800.x
van Wilgen, B.W., J.L. Nel, and M. Rouget. 2007. Invasive alien plants and South African rivers: A proposed approach to the prioritization of control operations. Freshwater Biology 52: 711–23. https://doi.org/10.1111/j.1365-2427.2006.01711.x
van Wyk, E., and B.W. van Wilgen. 2002. The cost of water hyacinth control in South Africa: A case study of three options. African Journal of Aquatic Science 27: 141–49. https://doi.org/10.2989/16085914.2002.9626585
Venter, F.J., R.J. Naiman, H.C. Biggs, et al. 2008. The evolution of conservation management philosophy: Science, environmental change and social adjustments in Kruger National Park. Ecosystems 11: 173–92. https://doi.org/10.1007/s10021-007-9116-x
Wallenfang, J., M. Finckh, J. Oldeland, et al, 2015. Impact of shifting cultivation on dense tropical woodlands in southeast Angola. Tropical Conservervation Science 8: 863–92. https://doi.org/10.1177/194008291500800402
Wegmann, M., L. Santini, B. Leutner, et al. 2014. Role of African protected areas in maintaining connectivity for large mammals. Philosophical Transactions of the Royal Society of London B 369: 20130193. https://doi.org/10.1098/rstb.2013.0193
Wetlands International. 2016. Mangrove restoration: To plant or not to plant? (Wageningen: Wetlands International). https://www.wetlands.org/publications/mangrove-restoration-to-plant-or-not-to-plant
Whitfield, A.K., G.C. Bate, T. Forbes, et al. 2013. Relinkage of the Mfolozi River to the St. Lucia estuary system—urgent imperative for the long-term management of a RAMSAR and World Heritage Site. Aquatic Ecosystem Health and Management 16: 104–10. https://doi.org/10.1080/14634988.2013.759081
Williams, A.E., R.E. Hecky, and H.C. Duthie. 2007. Water hyacinth decline across Lake Victoria—Was it caused by climatic perturbation or biological control? A reply. Aquatic Botany 87: 94–96. http://doi.org/10.1016/j.aquabot.2007.03.009
Wilson, J.R.U., P. Ivey, P. Manyama, et al. 2013. A new national unit for invasive species detection, assessment and eradication planning. South African Journal of Science 109: 0111. http://doi.org/10.1590/sajs.2013/20120111
Wilson, J.W., J.O. Sexton, R.R. Jobe, et al. 2013. The relative contribution of terrain, land cover, and vegetation structure indices to species distribution models. Biological Conservation 164: 170–76. https://doi.org/10.1016/j.biocon.2013.04.021
Zabbey, N., and F.B.G. Tanee. 2016. Assessment of asymmetric mangrove restoration trials in Ogoniland, Niger Delta, Nigeria: Lessons for future intervention. Ecological Restoration 34: 245–57. http://doi.org/10.3368/er.34.3.245
Zachariades, C., I.D. Paterson, L.W. Strathie, et al. 2017. Assessing the status of biological control as a management tool for suppression of invasive alien plants in South Africa. Bothalia 47: 1–19. http://dx.doi.org/10.4102/abc.v47i2.2142
Zalucki, M.P., M.D. Day, and J. Playford. 2007. Will biological control of Lantana camara ever succeed? Patterns, processes & prospects. Biological Control 42: 251–61. https://doi.org/10.1016/j.biocontrol.2007.06.002
Zarin, D.J., N.L. Harris, A. Baccini, et al. 2016. Can carbon emissions from tropical deforestation drop by 50% in 5 years? Global Change Biology 22: 1336–47. https://doi.org/10.1111/gcb.13153