3. What is Biodiversity?
© 2019 J.W. Wilson and R.B. Primack, CC BY 4.0 https://doi.org/10.11647/OBP.0177.03
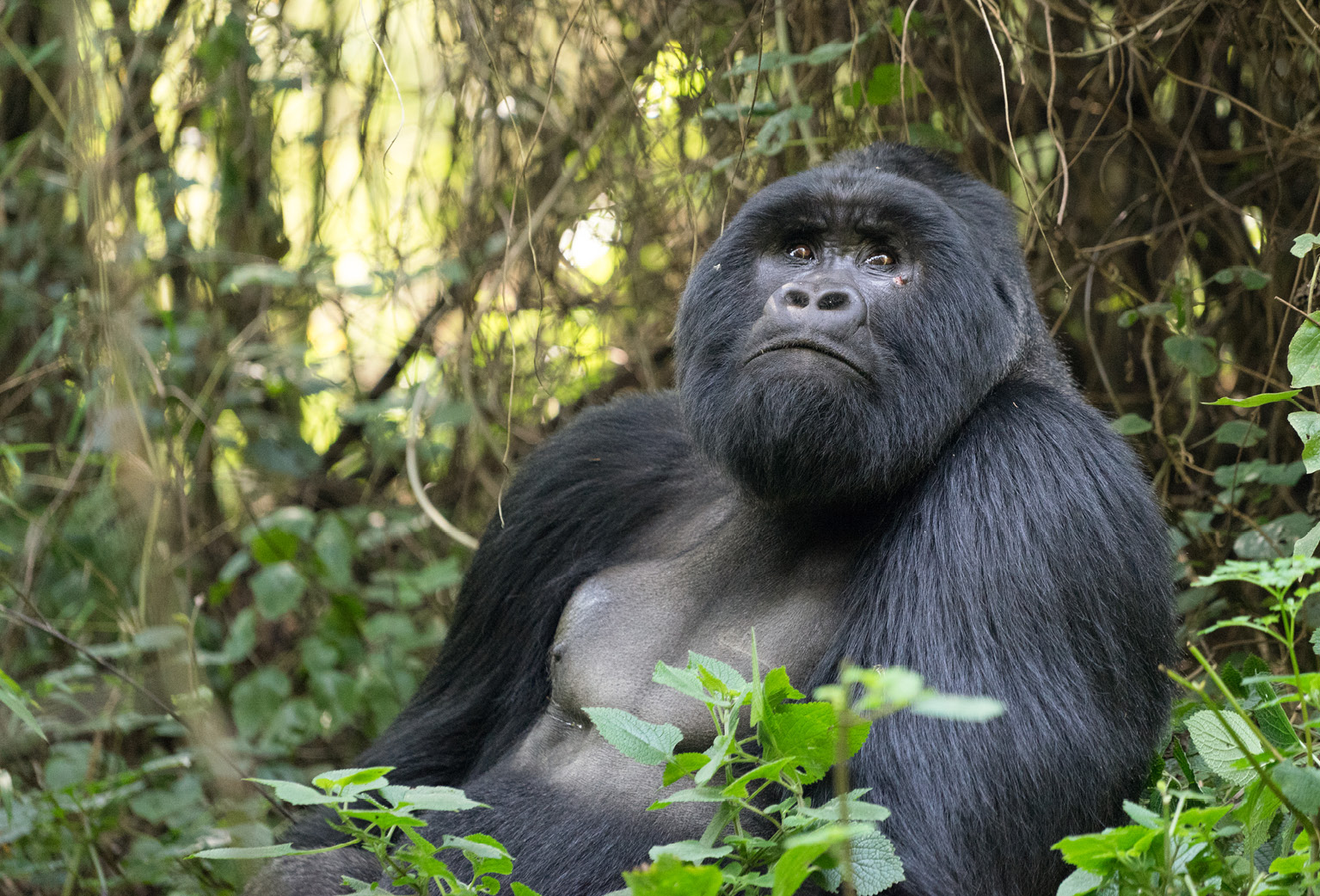
A “silverback” mountain gorilla (Gorilla beringei beringei, EN) in Mgahinga Gorilla National Park, Uganda. Once thought to be one species, genetic analyses have shown that there are two gorilla species, each with two subspecies. Thanks to the efforts of dedicated conservationists and local communities in the Albertine Rift, the IUCN downlisted the mountain gorilla from Critically Endangered to Endangered in 2018. The three other subspecies are still considered Critically Endangered. Photograph by Nina R, https://www.flickr.com/photos/150102727@N06/31467129021, CC BY 2.0.
Conservation biology aims to improve the protection of biodiversity—that is, all the species, genetic diversity, and ecosystems on Earth. By this definition, the process of documenting life on Earth requires us to consider biodiversity on three different levels (Figure 3.1):
- Species diversity: The full variety of species, from single-celled organisms like bacteria to larger multicellular organisms like animals and everything in between.
- Genetic diversity: The full range of variability in genetic material within a species. This variation can occur spatially as differences between populations or as differences between individuals of the same population.
- Ecosystem diversity: The full variety of ecosystems—i.e., assemblages of species and the physical environments in which they live.
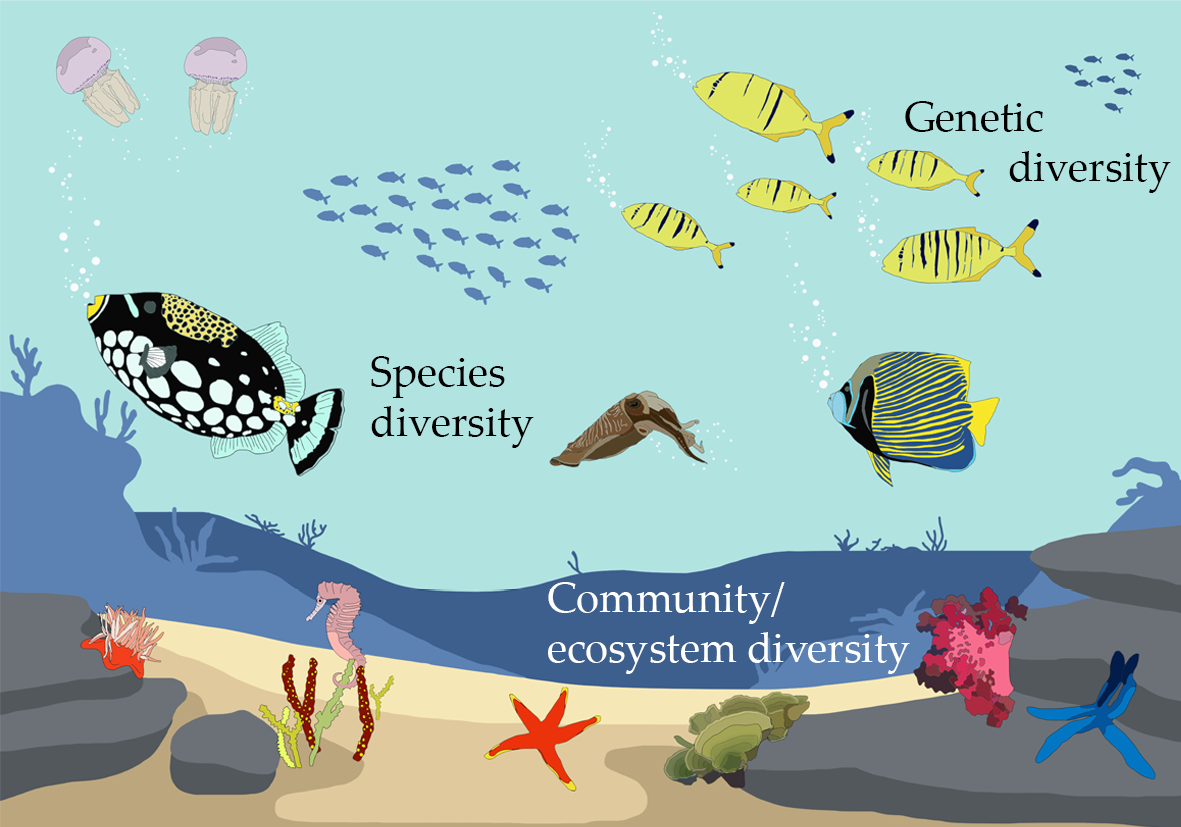
Figure 3.1 A region’s biodiversity includes the full complement of that area’s species diversity (all the area’s species), genetic diversity (the full range of genetic variation found within each of those species), and ecosystem diversity (the variety of ecosystems and ecological processes). CC BY 4.0.
The relationship between species, genetic, and ecosystem diversities is complex and interdependent. That is, a species cannot exist without genetic diversity or ecosystem diversity, and vice versa. For that reason, it is virtually impossible to affect one aspect of diversity without affecting the other. We can therefore think of species, genetic, and ecosystem diversities simply as different ways to measure the variety of life.
3.1 Species Diversity
In general, the first step in responding to the conservation need of a species or population is to know its identity. For this reason, one of the three main goals of conservation biology is to document all life on Earth or, in plain language, to give each species a name. The task of giving each species a (formal) name falls on specialist scientists known as taxonomists. Taxonomists (and the people assisting them) explore nature, collect specimens of plants, animals, and other organisms, describe/name those specimens, and store the specimens in permanent collections, such as natural history museums and herbaria (there are currently over 6,500 natural history museums in the world). These permanent collections, affectionally called “Libraries of Life”, provide the material and locations that taxonomists use to describe species and to develop systems for biodiversity classifications.
When a species is formally described, it is given a unique two-part name, known as a binomial name. For example, the binomial name for the lion is Panthera leo. The first part of the name, Panthera, identifies the generic epithet (or simply genus); in this case, the panthers or big cats. The second part of the name, leo, identifies a subset within the genus known as the specific epithet (or simply species); in this case, the lion. This binomial system thereby both identifies a lion as its own species and connects it to other closely-related species: Africa and Asia’s leopards (P. pardus, VU); Asia’s snow leopard (P. uncia, VU); Asia’s tigers (P. tigris, EN); and South America’s jaguars (P. onca, NT) (Figure 3.2).
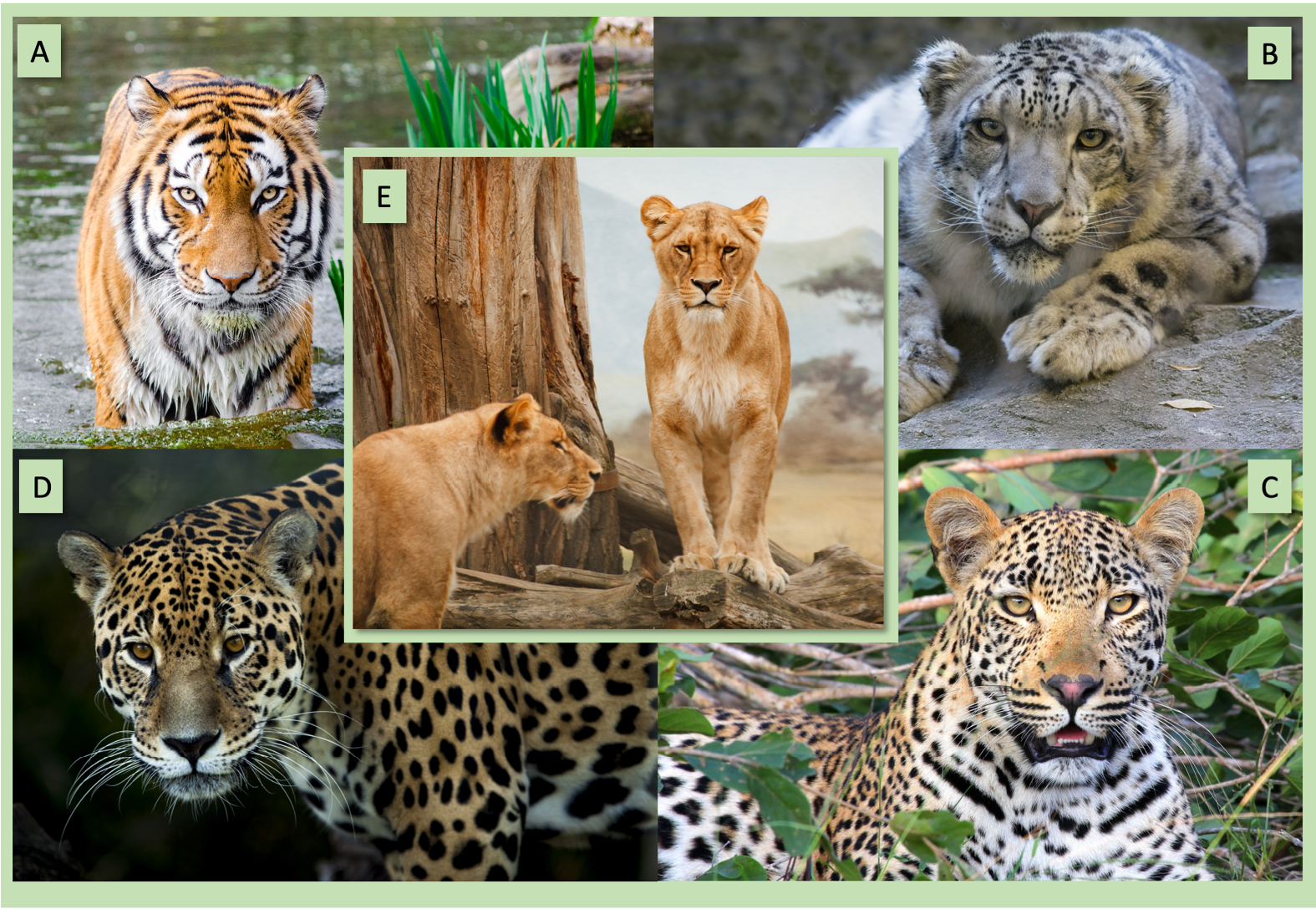
Figure 3.2 The world’s large predatory cats: (A) tiger, (B) slow leopard, (C) leopard, (D) jaguar, and (E) lion. By looking at their binomial names one can immediately see the five species are closely related. CC BY 4.0.
Binomial species names, as well as the taxonomic relationships between different species, form the backbone of taxonomic databases, as compiled and organised by biodiversity informatics projects. Some biodiversity informatics projects focus on one group of species, while others focus on certain regions. For example, all known marine species are listed in the World Register of Marine Species (http://www.marinespecies.org), while the Catalogue of Afrotropical Bees (https://doi.org/10.15468/u9ezbh) collates information of only African bees. In some cases, multiple projects—each using different assumptions to suit different user groups better—may catalogue the same group of species. For example, the world’s fungi, are listed both in Index Fungorum (http://www.indexfungorum.org) and MycoBank (http://www.mycobank.org), while bird names are indexed by at least seven different projects, each a little different from the other. There are even some biodiversity informatics projects that attempt to catalogue all life on Earth; examples include Catalogue of Life (http://www.catalogueoflife.org), Encyclopaedia of Life (http://eol.org), and Wikispecies (https://species.wikimedia.org).
3.1.1 What is a species?
There are three rules of thumb that taxonomists use to describe a species:
- Morphological definition of species: Individuals that are distinct from other groups in their morphology, physiology, or biochemistry.
- Biological definition of species: Individuals that breed (or could breed) with each other in the wild, but do not breed with members of other groups.
- Evolutionary definition of a species: Individuals that share a common evolutionary past, usually indicated by genetic similarities.
In practice, conservation biologists generally rely on the morphological definition to identify species. The ability to recognise physical or morphological differences between organisms is handy even when the actual identity of specimens is unknown. In such cases, field biologists may refer to the unknown species as morphospecies (Figure 3.3), at least until an expert identifies the unknown individuals or a taxonomist gives them an official scientific name. In contrast, the biological definition of species relies on information that is difficult to obtain and thus not readily available. The biological definition also fails to recognise recent speciation, which can cause closely related but distinct species to interbreed. Similarly, it is generally impractical for fieldworkers to measure differences in genetic sequences to distinguish one species from another because these procedures currently require expensive, immovable laboratory equipment.
Taxonomists can use morphological, biological, and genetic information to identify species.
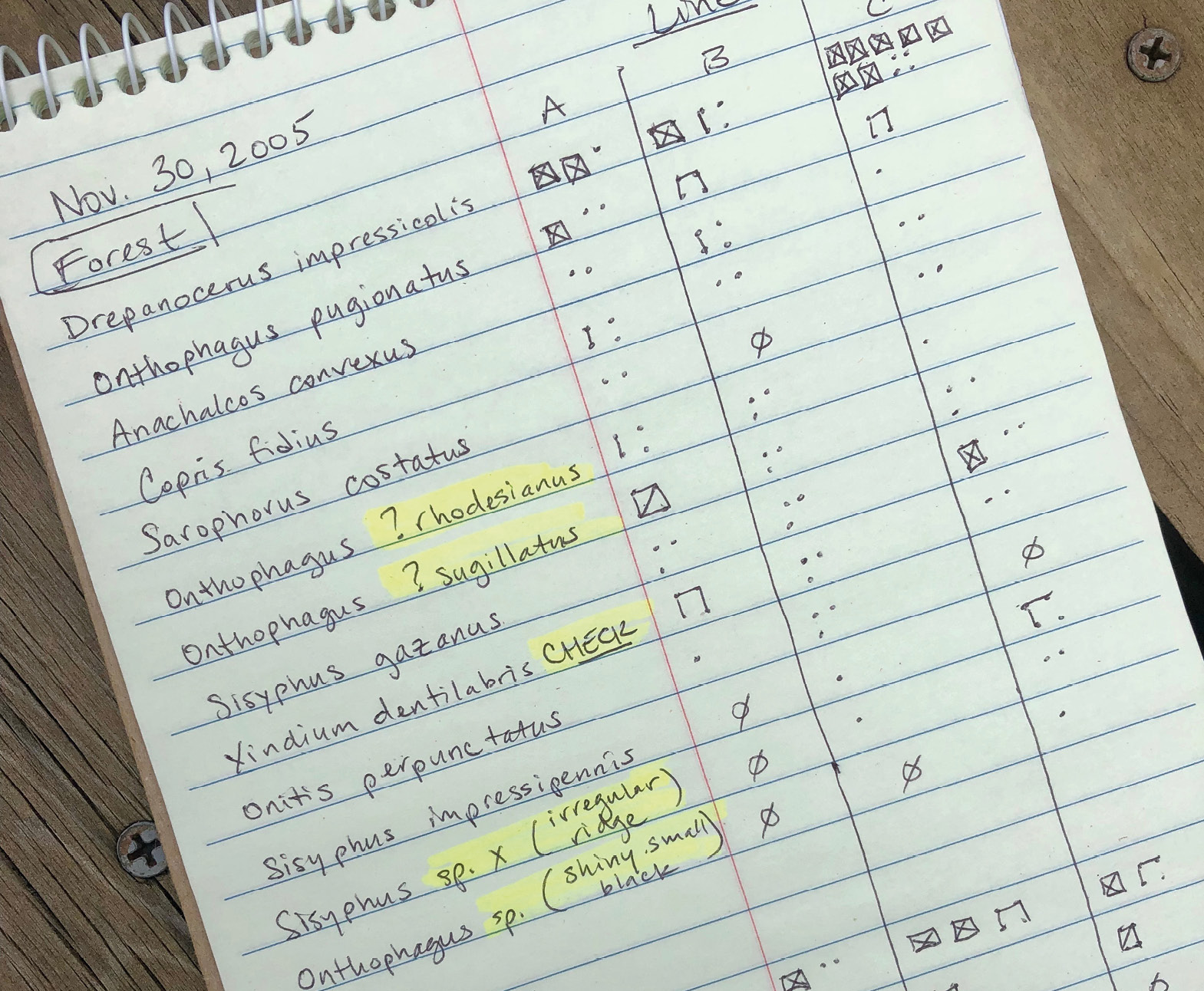
Figure 3.3 Field sheet showing application of the morphospecies concept during a dung beetle surveys in South Africa. Unidentified (and potentially misidentified) specimens are noted with a variety of descriptors (highlighted) and collected for identification at a later stage. Photograph by Lesley Starke, CC BY 4.0.
Despite the practical difficulties of applying the biological and evolutionary definitions in the field, both provide important guidelines for conservation efforts. The biological species definition allows us to better understand species biogeography and the mechanisms that prevent two closely-related species to interbreed. The evolutionary species definition in turn allows us to better understand how and why the genetic makeup of populations change over time, through processes such as random mutations, natural selection, emigration, and immigration. It is thus important for conservation biologists to acknowledge the importance of maintaining these dynamic processes in protecting natural systems, and where possible, include them in their fieldwork (Box 3.1).
Box 3.1 Finding a Needle in a Haystack: Monitoring Species Using eDNA
Centre for Invasion Biology, Stellenbosch University,
Stellenbosch, South Africa.
trobins@sun.ac.za , clovamabin@gmail.com
Trying to find threatened species in aquatic systems can be like trying to find a needle in a haystack. Traditionally, researchers have set off with nets, buckets, and even snorkels and scuba gear to painstakingly search for threatened species in ecosystems, ranging from streams to coral reefs. While searching in a small system, such as a pond, might not seem too difficult, it can be a real challenge to find tiny, inconspicuous organisms in and amongst the mud, stones, and plants, especially when they are trying their best to remain hidden. Things get even trickier when combing through large ecosystems like lakes or bays. These difficulties make it hard to reliably monitor the status or distribution of threatened aquatic species.
However, scientists have recently developed a new search tool called environmental DNA, (eDNA in short), where researchers collect and search water samples for the DNA of the species they are interested in. The eDNA technique was first developed by a biologist trying to detect organisms in sediment (Willerslev et al., 2003) but is now being used by conservationists working in all kinds of aquatic ecosystems. Organisms continually release small amounts of DNA into the water by sloughing off skin or other cells and releasing bodily wastes. This DNA then mixes in the surrounding environment, allowing those organisms to be detected through genetic analyses without actually sampling them directly.
Researchers have been testing just how useful eDNA is for finding threatened species in ponds and streams (Thomsen et al., 2012). They detected the eDNA of fish, shrimp, dragonflies, and amphibians in most ponds where the species were known to occur and found no trace of the eDNA of these species where they were absent. The most exciting development was their ability to detect eDNA evidence of threatened species in places where they had previously occurred but not been recently recorded by traditional search methods. Field observations and experiments also showed that eDNA can persist for up to two weeks in fresh water, and that concentrations can correspond to population sizes; this suggests that scientists may be able to monitor the abundance of rare aquatic species to a high degree of accuracy using this approach. For example, Lake Victoria could be searched for rare cichlid fish species that may still be present at low numbers even though researchers have not seen them for several years.
eDNA technology also holds considerable promise for the management of aquatic invasive species, if they could be detected as new arrivals before their numbers grow enough to be detected by conventional methods (Takahara et al., 2013). Early detection will give conservation managers a head-start and enable them to react quickly to invasions and increase their chances of preventing the environmental damage associated with invasive species. In a local twist to the tale, ongoing work in South Africa is applying eDNA as a tool for measuring the success of management efforts aimed at removing the invasive marine European shore crab (Carcinus maenas) (Figure 3.A) that could outcompete or threaten native African marine species. It is hoped that eDNA will be able to track the decline in crab numbers as the species is removed and then be used to monitor for any new arrivals should the crabs re-invade.
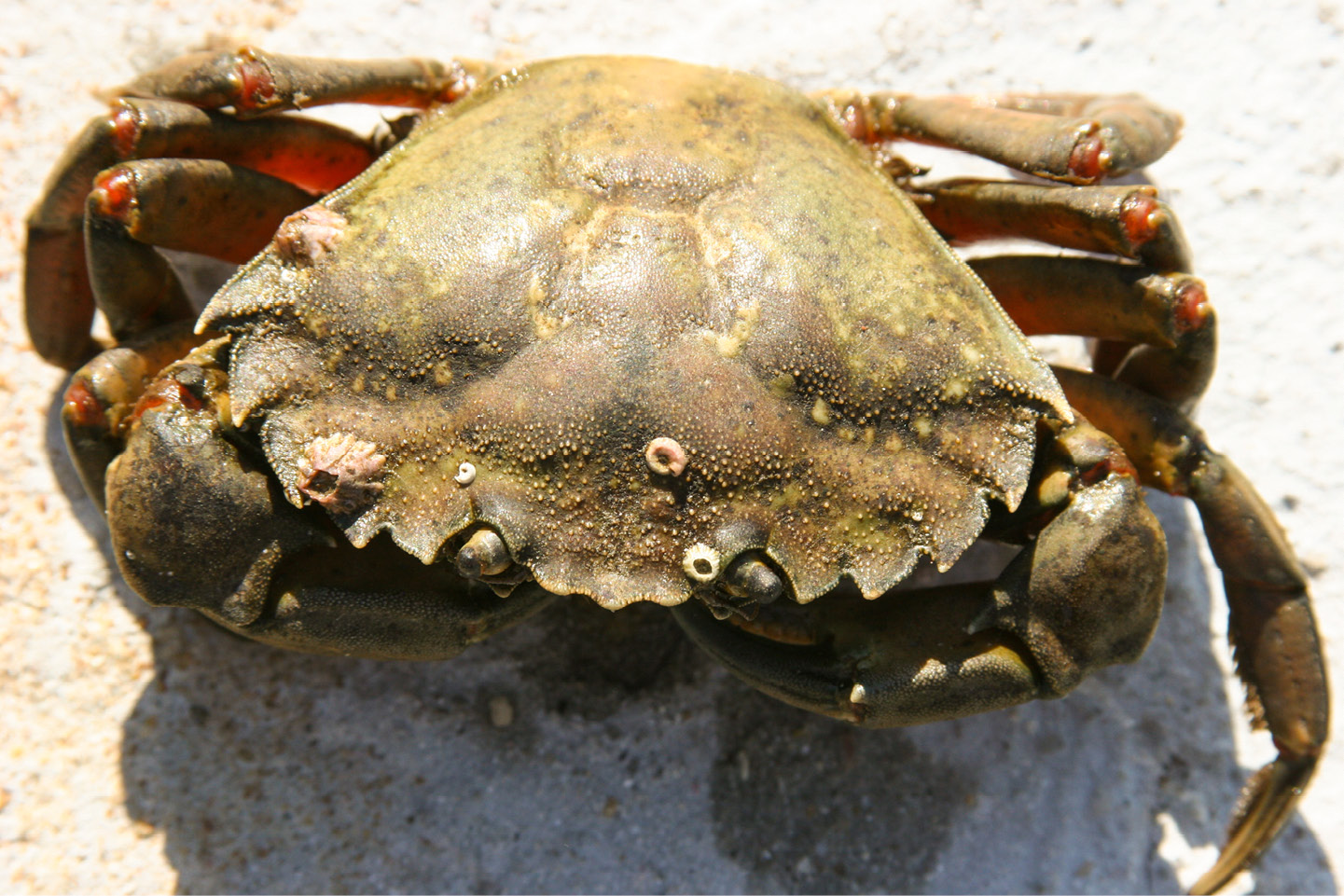
Figure 3.A Researchers in South Africa have been using eDNA to monitor the success of a control programme aimed at invasive European shore crabs. Photograph by Clova Mabin, CC BY 4.0.
This exciting new approach in detecting species is rapidly developing and improving our efficiency at monitoring threatened and invasive species. This makes the process less like looking for a needle in a haystack, and more like finding the millions of needles right under your nose.
3.2 Genetic Diversity
Every extant species on Earth consists of at least one population, a group of individuals at a certain place that generally look alike and can potentially breed with each other to produce offspring. These population(s) can be very small (just a few individuals), very large (billions of individuals), or anything in-between. The individuals within each population generally differ genetically from one another to some degree. This genetic variation, a component of genetic diversity (Figure 3.4), exists because the genes—the functional units of hereditary information that provide the blueprint of an organism—in different individuals are made up of slightly different DNA sequences. Different forms of a gene, which arise through mutations that change DNA sequences, are known as alleles. The gene pool, in turn, consists of the total diversity of genes and alleles in a population or species. The particular mix of genes and alleles in an individual is its genotype. The expression of an individual’s genotype, as determined by the environment where an organism has developed, is its phenotype—that is, the organism’s morphology, anatomy, physiology, and biochemistry. Common characteristics to describe a person include height, hair colour, and blood type, which taken together begin to describe that person’s phenotype.
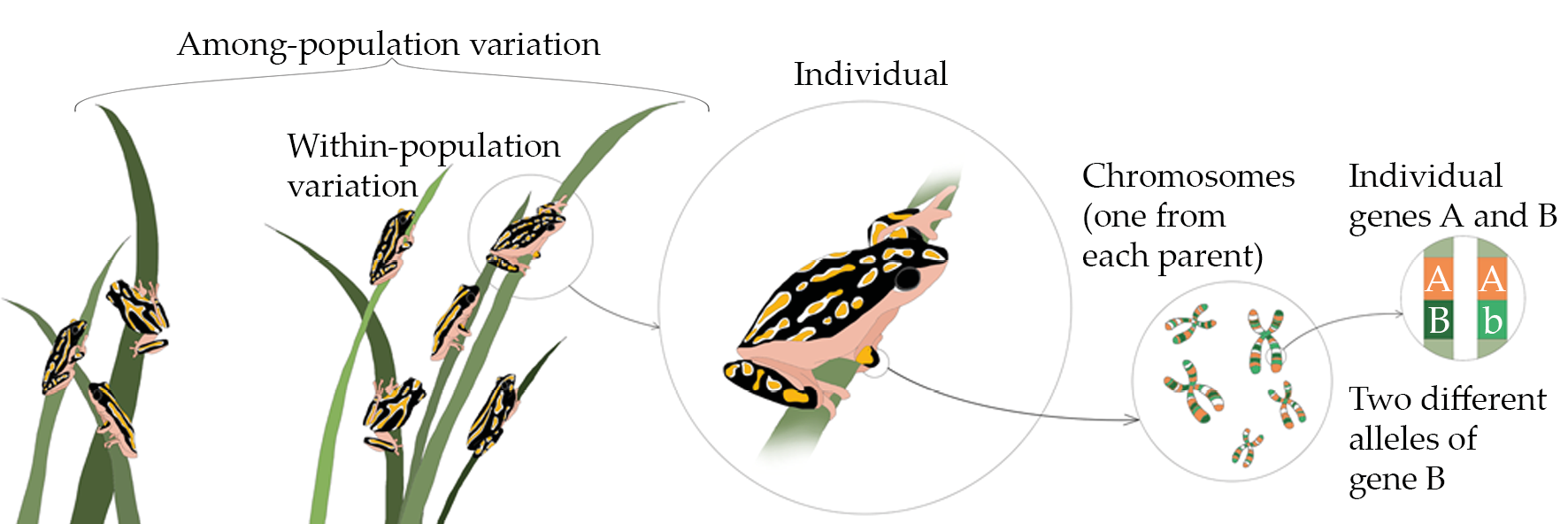
Figure 3.4 Genetic diversity arises due to variation in the alleles of individual genes and variation in chromosomes from different parents, which give rise to genetic variation between individuals, both within the same population and between different populations. CC BY 4.0.
In species which reproduce asexually, the potential for increased genetic diversity is limited to DNA mutations. However, sexual reproduction creates new genetic combinations by bringing together chromosomes from each parent. This process, called recombination, results in offspring that are genetically unique from their parents. Genetic mutations provide the foundation of genetic variation, but sexual reproduction dramatically increases genetic diversity by randomly mixing alleles in different combinations.
Genetic diversity enables species to adapt to environmental change.
Two factors determine a species’ genetic diversity: the number of genes that have multiple alleles (polymorphic genes) and the number of alleles present in a population for each polymorphic gene. If a gene is polymorphic, some individuals will have two different forms of the gene—that is, they will be heterozygous because they received different alleles of the same gene from their parents. Some individuals will have two of the same forms of the gene—they will be homozygous because each parent gave them the same allele. In general, the greater the genetic diversity in a population, especially the greater number of alleles present, the more capable a species will be to adapt to changing circumstances in their environment. Genetics also affect an individual organism’s development, physiology, and fitness—the relative ability of individuals to survive and reproduce. This same principle gives humans the ability to select and breed crops and domestic animals with characteristics that benefit the production and quality of food (Davis et al., 2012). Many rare species have relatively low genetic diversity, especially in populations which have dwindled to small sizes. Low genetic diversity limits small populations’ ability to adapt to changes in environmental conditions and leaves them at risk of extinction when conditions do change. Section 8.7 discusses the importance of maintaining genetic diversity in greater detail.
3.3 Ecosystem Diversity
Those who have climbed Africa’s highest mountains have likely noticed how the plants and animals present gradually change, as one moves from tall lowland forest to moist, mid-elevation forest with a low canopy, then into grassy alpine meadows, and lastly, onto cold, windy, and rocky mountain peaks. We see these changes because, as we move across the landscape, physical conditions (e.g. geology, soil type, temperature, precipitation) change, and so also the species adapted to different environmental niches, as determined by the varying conditions. Thus, one by one, the species present at one location are replaced by new species better suited to the new conditions. We can see how the whole landscape changes in response to dynamic biotic and abiotic components of the environment (Figure 3.5). The variety of life resulting from these environmental changes is what gives rise to ecosystem diversity.
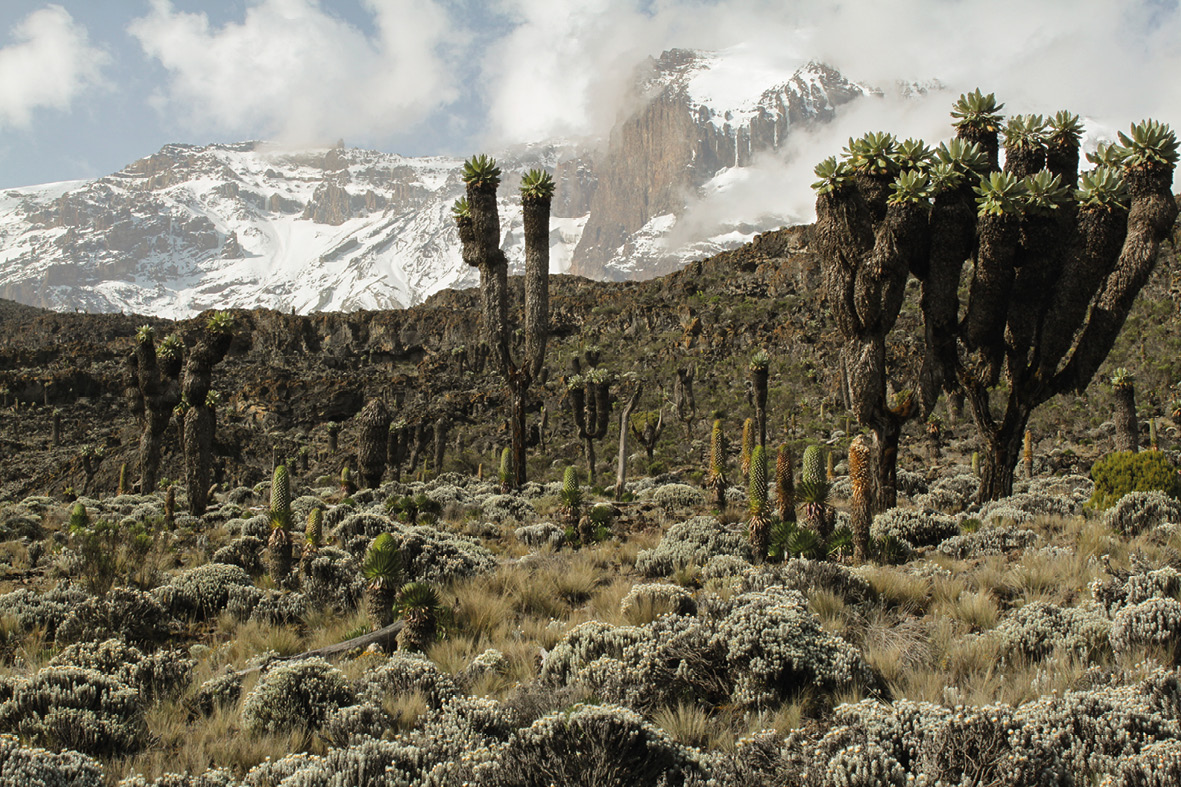
Figure 3.5 Climate plays an important role in the distribution of biodiversity. That is why we see a gradual decline in species diversity as one moves from warm and humid lowlands towards cold and windy peaks of high mountains. This photo was taken on Mount Kilimanjaro in Tanzania around 3,800 m above sea level. Photograph by Andreas Ensslin, CC BY 4.0.
Ecosystem diversity describes the full variety of ecosystems of an area, while the term “ecosystem” describes all the organisms in an area, as well as the physical and chemical environment with which those organisms interact. An important component of any ecosystem is its biological community (or ecological community), defined as all the living individuals, populations, and species of a place, as well as all the biological interactions among those organisms. The abiotic (or physical) environment, especially climate, energy, and nutrients availability, greatly affects the structure, composition, and characteristics of an area’s biological community (or biotic environment), and ultimately the type of ecosystem present (Figure 3.6). For example, water that evaporates from leaves, the ground, and other surfaces may later become rain or snow that provides drinking water that sustains life. Sunlight energy, in turn, enables photosynthetic plants (or primary producers) to grow; the energy from the sun is later transferred to animals that eat the plants (herbivores, or primary consumers), and then to animals that eat other animals (carnivores, or secondary consumers). The physical environment similarly affects aquatic ecosystems. For example, in freshwater stream, the biological community present is determined in large part by the physical characteristics of the stream, including water chemistry, temperature, flow rate, and substrate.
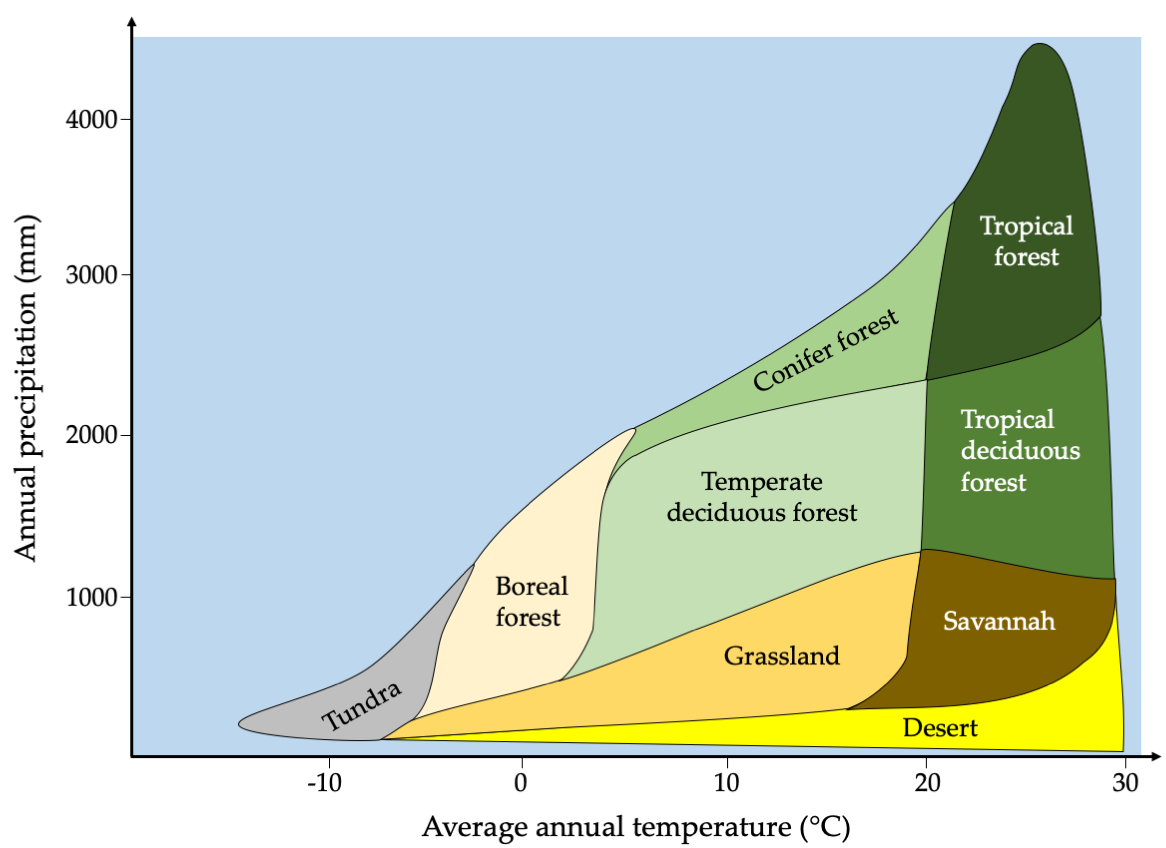
Figure 3.6 An area’s abiotic components strong influence its biotic environment. For example, average temperature and precipitation determine which biome will dominate, which in turn influences which species will be present. After Whittaker, 1975, CC BY 4.0
At local scales, biological communities themselves can play prominent roles in altering the physical environment. For example, the trees present in a forest ecosystem can influence wind speed, light, humidity, soil chemistry, and temperature. Likewise, marine biological communities, such as kelp forests, seagrass beds, and coral reefs, can affect water temperature, water chemistry, sunlight penetration, and wave energy.
Within a biological community, individual species have specific ecological roles and have different requirements for survival. These roles and requirements enable different species to coexist, and in cases of interdependency, necessitate that they do so. For example, a given plant species may grow only in one type of soil, be pollinated by one type of insect, or have its seeds dispersed by only one type of animal. If any one of these requirements restricts the population size or distribution of that plant, it is considered a limiting resource. Even animal dung, usually considered a waste product, may become a limiting resource to species that rely on it for feeding and breeding. For example, studies from Côte d’Ivoire and Southern Africa have linked dung beetle population declines to the extirpation of large herbivores such as elephants and buffaloes (Nichols et al., 2009).
Environmental conditions that regulate the abundance of limiting resources may change over time. Consequently, many ecological communities can undergo major shifts in their composition over time. This is particularly prominent during ecological succession, which describes the gradual process during which ecosystems change after a disturbance. Consider, for example, an old-growth forest that is cleared by a logging operation. Shortly after clearing and abandonment, the soil absorbs more sunlight, resulting in high temperatures and low humidity during the day. These early stages present an ideal environment for pioneer species, such as sun-loving butterflies, annual herbs, and grasses, with wind-dispersed seeds. In a few years’ time, the early successional herb-field or grassland transition to a scrubland, which accommodates a new suite of species. As the shrubs mature, forest trees germinate in the shade provided by the shrubs. Over the course of decades, as the forest trees mature, the forest canopy is gradually re-established which, in turn, provide opportunities for species characteristic of mid- and late-successional stages, such as shade-tolerant wildflowers of moist soils. Eventually, after many decades, climax species representative of mature forests, such as birds that nest in the holes of dead trees, start colonising the area.
3.4 Patterns of Biodiversity
Developing a strategy to conserve biodiversity requires a firm understanding of where threatened species and populations occur, why they are threatened, what their needs are, and what role they play in their respective ecosystems. By obtaining an understanding of species’ distributions, biologists simultaneously gain an initial rough “estimate” of genetic diversity and ecosystem diversity. While addressing these questions is a critical task, finding appropriate answers can be complex, expensive, and take a long time to solve. This is in no small part because identifying species can, at times, be a very challenging endeavour.
3.4.1 Challenging species identifications
Before biologists can determine a species’ distribution, needs, and population status, it is important to know the identity of the individuals being studied. While this may sound like a straightforward task, the process of identifying (and naming) a species can be deceptively hard, even for professional taxonomist. For example, a recent study found that 58% of 4,500 wild African ginger (Aframomum spp.) specimens that were deposited by professional biologists across 40 herbaria in 21 countries were given the wrong name (Goodwin et al., 2015)!
Describing species can be difficult, in part, because the multiple methods used by biologists to separate species do not always give the same results.
Identifying species can be hard, in part because the three tests biologists use to separate different species—morphology, biology, and evolution—do not always give the same results. That is because the methods and assumptions of each test are different. For example, some species have several varieties with easily observed morphological differences but are biologically and genetically similar enough that all those varieties are still considered a single species. A well-known example is the single species Canis familiaris, or domestic dog, whose wildly variable and numerous breeds can interbreed despite their large morphological differences. In contrast, some butterflies are considered distinct species because they cannot interbreed and have a characteristic genetic makeup, even though they cannot be separated by the naked eye.
Another important aspect complicating species identifications is that speciation—whereby one species evolves into another—is a slow and gradual process; for some species, it may take many thousands of years. Consequently, much controversy exists about where to draw the “new species” line; in other words, when is a species distinct enough to be considered a separate species? Africa’s iconic giraffes (Giraffa camelopardalis, VU) are a case in point. Taxonomists recently suggested that the region’s giraffes—previously considered a single species—may, in fact, consist of four (Fennessy et al., 2016) or even six (Brown et al., 2007) species. Unfortunately, the final number of giraffe species is still disputed because of the different assumptions made by each study and how that impacts the number of species (Bercovitch et al., 2017). Similarly, biologists often struggle to split and identify cryptic species—undescribed species that are wrongly grouped with other similar-appearing species. A recent study estimated that 60% of newly discovered species are cryptic (Ceballos and Ehrlich, 2009). Even well-known groups may suffer from this problem: there is a reasonable chance that the bushbuck (Tragelaphus scriptus. LC) and klipspringer (Oreotragus oreotragus, LC) may in fact consist of several cryptic species yet to be described (Plumptre and Wronski, 2013; Groves et al., 2017).
Hybridisation plays an important role in speciation, but it can also be detrimental to conservation efforts, particularly when it involves rare species and/or human disturbance.
To complicate matters even further, some species are closely related enough that they sometimes mate and produce hybrids. These hybrids blur the distinction between species, particularly those that may be early in the process of speciation. For some taxa, hybridisation naturally occurs in areas where the distribution ranges of related species overlap (e.g. de Jong and Butynski, 2010). Such natural hybridisation plays an important role in speciation (the evolution of new species); for example, it may have contributed to the high diversity of cichlid fishes in Africa’s Rift Valley lakes (Salzburger et al., 2002). But hybridisation can also be detrimental to conservation efforts, particularly when it involves rare species and/or human disturbance. For example, when humans reduce one species’ populations so much that they struggle to find reproductive partners of their own kind (e.g. vaz Pinto et al., 2016), when humans remove dispersal barriers that kept related species apart (e.g. Mondol et al., 2015), or when humans force related species that naturally occupy separate distributions to live together through translocations (e.g. Grobler et al., 2011; Benjamin-Fink and Reilly, 2017; van Wyk et al., 2017). While some hybrids may be sterile and thus unable to reproduce, at other times the resulting offspring can be quite strong in an evolutionary sense—a condition known as hybrid vigour (or heterosis)—and may outcompete their parent species. Such is the case with a land snail from the Seychelles (Pachnodus velutinus, EX), which was recently driven to extinction by hybridisation with a closely-related species (Gerlach, 2009)—hybrid individuals can still be found where P. valutinus used to occur.
Conversely, there may also be times when conservation biologists get it wrong and prioritise a species that does not warrant specific status. The Liberian greenbul (Phyllastrephus leucolepis) is one such example. Known from only a handful of records, this species was considered Critically Endangered until 2016, when geneticists discovered that the Liberian greenbul was the same species as the common icterine greenbul (Phyllastrephus icterinus, LC), but with an unusual coloration due to nutrient deficiencies (Collinson et al., 2018).
3.4.2 Implications of challenging species identifications
The difficulties in distinguishing between species have several practical conservation implications. First, when it is hard to identify a species, it may also be hard to determine that species’ true population size and distribution which, in turn, impacts its conservation status. This was illustrated in a study on bushmeat markets in Guinea-Bissau, which showed how primate misidentifications hide the true impact of hunting on some of the region’s most impacted species (Minhós et al., 2013). It also hampers captive breeding projects, by making the captive populations susceptible to outbreeding depression, which occurs when individuals that are not closely related (i.e. from different populations) breed and produce offspring (Conservation genetics is discussed in more detail in Section 8.7). Lastly, identification challenges with cryptic species can also cause delays in the formal description process, a necessary step in writing effective laws to protect them. The recent controversy among biologists arguing whether Africa’s elephants are one or two species is a case in point. African elephants were already considered threatened when biologists thought they were a single species. This all changed in 2005, when taxonomic authorities officially recognised two elephant species, in effect dividing a single threatened species into two (thus even more imperilled) species (CBD, 2015). Yet, to avoid leaving hybrid elephants (e.g. Mondol et al., 2015) with an uncertain conservation status, the IUCN continues to assess elephants as one single species (Blanc, 2008); thus, their current Vulnerable assessment may not be an accurate reflection of each species’ true conservation status.
Despite these challenges, conservationist biologists need to make every effort to obtain correct identifications. For most studies, morphological methods may be adequate. But when there is doubt, it is important for researchers to confirm their identifications with additional methods. Recent progress in making genetic technology more widely accessible through hand-held devices (Pennisi, 2016; Parker et al., 2017) and techniques such as DNA barcoding has also greatly enhanced our ability to correctly classify cryptic species, allowing us to give those species the conservation attention they deserve (Box 3.2).
Box 3.2 Golden Mole Conservation Requires a Sound Taxonomy
1Department of Genetics, &
2Department of Zoology and Entomology,
University of Pretoria, South Africa.
smaree@zoology.up.ac.za , samantha.mynhardt@up.ac.za
Golden moles (Chrysochloridae) are small, subterranean insectivores that rank among Africa’s most unique, most threatened, and yet poorly studied mammals thanks to their secretive burrowing lifestyle. Ten of the 21 known species are currently threatened with extinction (IUCN, 2019) as their highly restricted and naturally fragmented sandy soil habitats are under threat from human activities. Current conservation efforts are severely jeopardised by taxonomic uncertainties and ambiguous evolutionary relationships, thus far based on morphological and limited genetic data, which suggest that many distinct but cryptic species remain undescribed (Taylor et al., 2018).
To remedy the dearth in knowledge on two endemic South African golden mole species, we analysed molecular data of individuals collected across the entire distribution range of both Juliana’s golden mole (Neamblysomus julianae, EN) and Hottentot golden mole (Amblysomus hottentotus, LC) (Figure 3.B). In contrast to the widespread Hottentot golden mole, the Juliana’s golden mole counts among South Africa’s most imperilled mammals and is known from only three range-restricted, geographically isolated populations (Maree, 2015, Maree et al., 2016; Maree, 2017; Taylor et al., 2018). These three populations, together covering less than 160 km2 occur in southeastern Pretoria (Gauteng population), the district of Modimolle (Limpopo, ~ 120 km north of Pretoria), and in southwestern Kruger National Park (Mpumalanga, ~ 400 km east of Pretoria) (Figure 3.C).
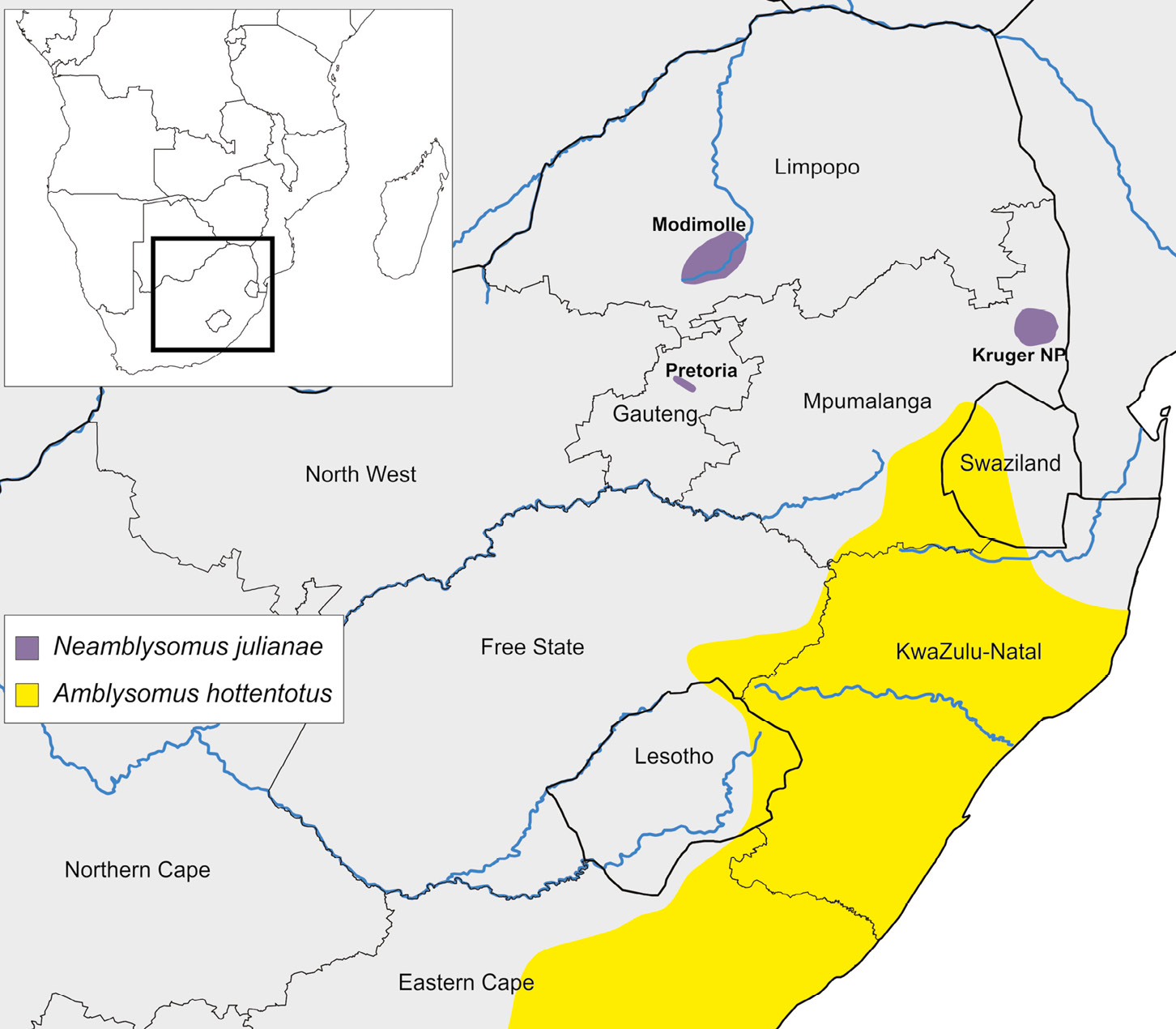
Figure 3.B The known geographic distribution of the widespread Hottentot golden mole and range-restricted Juliana’s golden mole. Map by Arrie Klopper, after IUCN, 2019, CC BY 4.0.
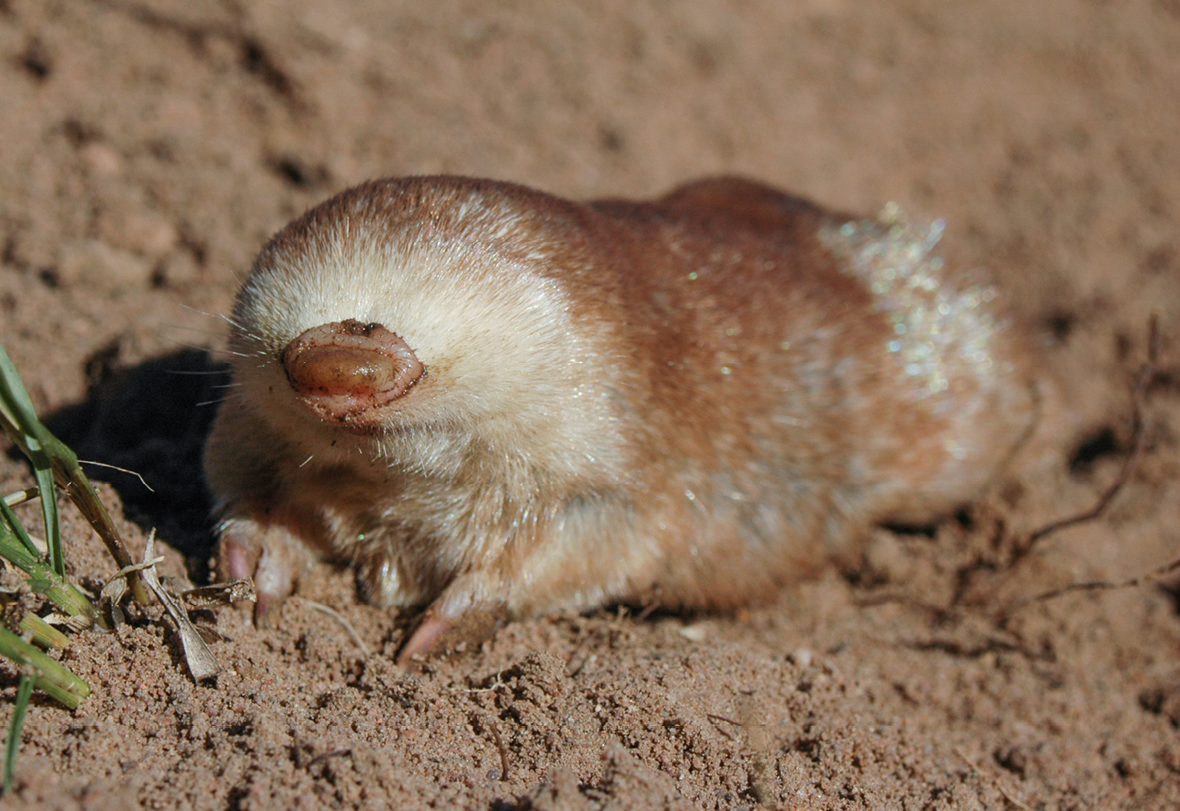
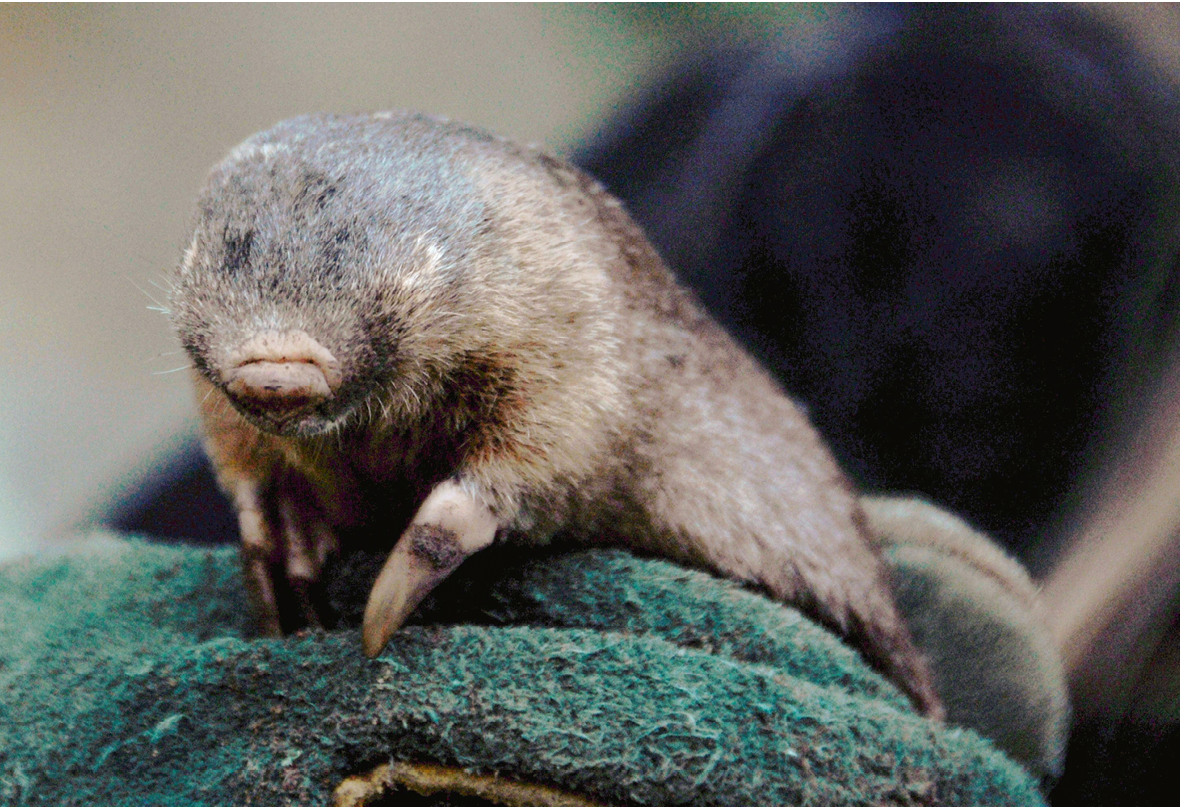
Figure 3.C (Top) The Juliana’s golden mole is one of Africa’s most threatened mammals. Photograph by Craig R. Jackson, CC BY 4.0. (Bottom) The Hottentot golden mole is generally thought of as widespread across southeastern South Africa but may in fact consist of several undescribed cryptic and potentially threatened species. Photograph by Samantha Mynhardt, CC BY 4.0.
Using molecular and other genetic methods, we have gained insights about the evolutionary relationships and gene flow between these two golden mole species, which have several conservation implications. First, preliminary findings suggest that the Hottentot golden mole contains several morphologically similar, but evolutionary distinct and genetically divergent lineages, some of which would represent undescribed cryptic species (Mynhardt et al., 2015; Taylor et al., 2018). Similarly, preliminary evidence suggests the Juliana’s golden mole contains pronounced genetic separation between the Mpumalanga population and the Gauteng and Limpopo populations. This also corresponds to morphological differences observed between these populations, which collectively suggest that the Mpumalanga population of Juliana’s golden mole might in fact be a cryptic species (Maree, 2015; Maree et al., 2016; Maree, 2017; Taylor et al., 2018). Unfortunately, in each of these cases the knowledge gaps remaining precluded definitive conclusions. Rigorous geographic sampling and additional molecular/genomic analyses will be needed to confirm the taxonomic status and geographic boundaries of putative new species within these and other golden mole taxa (Taylor et al., 2018).
Our results show that genetic frameworks contribute substantially to informed conservation decision-making. For golden moles and other taxa, some newly described species will undoubtedly be considered more threatened than in their previous species designations. Threat assessments on the Juliana’s golden mole has already identified the Gauteng population as Critically Endangered due to severe habitat loss and transformation within its highly restricted and already fragmented range (~ 22 km2 in extent) caused by rapid urbanisation and opencast sand mining. This pressure is exacerbated by this species’ extreme habitat specificity and poor dispersal capabilities (Jackson and Robertson, 2011; Maree, 2015; Maree et al., 2016; Maree, 2017; Taylor et al., 2018). Species distribution modelling (SDM, discussed in Section 10.1.1) predicted several regions throughout Gauteng, Mpumalanga, and Limpopo Provinces where the species could potentially occur, but subsequent surveys led to the discovery of only two new localities around Modimolle (Jackson and Robertson, 2011). This finding emphasises that the protection of all suitable habitats remaining for the species and the Pretoria population, in particular, would be key to its persistence. Strategies to achieve this ought to be incorporated into current conservation planning (Maree, 2015; Maree et al., 2016; Maree, 2017; Taylor et al., 2018).
We also illustrated the importance of maintaining the integrity of geographically isolated and/or genetically unique populations, lest yet undescribed species be lost to extinction before they could be fully recognised. A sound taxonomy, obtained through genetic analyses, thus contributes substantially to informed conservation decision-making. Even in the absence of such information, it is still crucial that isolated populations be managed as distinct units to conserve the evolutionary history of different species and populations.
Because the demand for expert taxonomists outstrips their availability, there is also a need to train and employ more taxonomists, particularly in the tropics and other species-rich areas. The public can help in this endeavour. In 2015, citizen scientists—volunteers participating in scientific projects—discovered 51 of 60 new dragonfly species from Africa that were described that year (Dijkstra et al., 2015). For conservation biologists, it is also important to not become despondent about the lagging efforts to describe species. They should instead take an example from motivated parrot lovers who were motivated to work even harder to get their study species recognised as distinct (Box 3.3). It is also important to keep in mind that species are never fixed; evolve all the time, albeit at different rates, due to challenges and opportunities presented by their environment.
Box 3.3 Does Tardy Recognition of a Species Hamper its Conservation?
School of Life Sciences, University of KwaZulu-Natal,
Pietermaritzburg, South Africa.
downs@ukzn.ac.za
The usefulness of subspecies in conservation has long been a subject of controversy (Coetzer et al., 2015). Accurately drawing the line between an individual species and other similar animals is important for effective studies of biodiversity, and for planning and implementing official conservation strategies. Across Africa, there are many species with very broad historical distributions that are thought to contain locally adapted varieties. However, the distributions of many of these species are now fragmented and disjointed, mainly because of changes in available habitat. Examples include reptiles, such as the Nile crocodile (Crocodylus niloticus, LC), mammals, such as the common hippopotamus (Hippopotamus amphibious, VU), and a wide range of bird species. As a result of this fragmentation, various subspecies, recognised by morphology and habitat distribution, are now recognised as individual species. Modern DNA technology allows these discoveries to be supported with genetic evidence.
Protecting a newly recognised species can be difficult; genetic testing takes time and funding, and if an animal or plant is threatened before it has full species status, conservation success is that much more difficult. An example is the Cape parrot (Poicephalus robustus, EN), a forest species which was first suggested in 1997 to be a separate species and distinct from the more widespread grey-headed parrot (Poicephalus fuscicollis, LC) of Africa’s savannah ecosystems. Additional support for the Cape parrot (Figure 3.D) being a separate species came from ecological and morphological data in 2002 (Wirminghaus et al., 2002) and separate genetic evidence in 2015 (Coetzer et al., 2015). Although many published bird guides reflect the change, the species was recently recognised as a species by authorities (e.g. BirdLife International, 2017), which affected its ability to receive legal protection. The Cape parrot is endemic to South Africa, with a distribution primarily restricted to southern mist-belt Afromontane forests in the Eastern Cape and southern KwaZulu-Natal plus a relict population in Limpopo Province. Cape parrots are restricted in their distribution by their specialised habitat and dietary requirements for particular fruits. A decrease in this species’ abundance over the past 50 years is a consequence of several factors, including habitat fragmentation and degradation, food and nest site shortages, illegal trade of the birds for pets and aviculture, and disease.
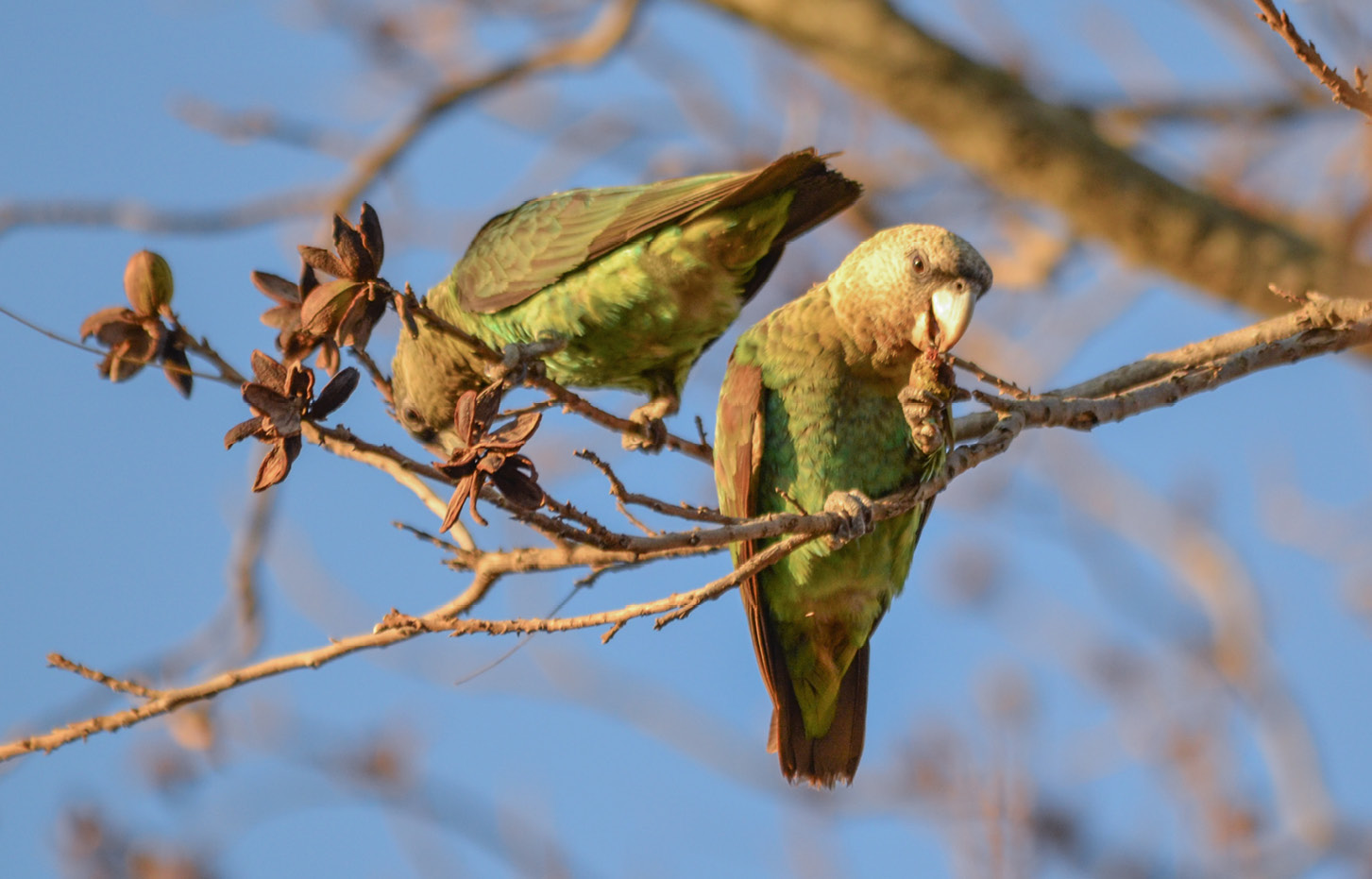
Figure 3.D Juvenile Cape parrots feeding on pecan nuts near Creighton, KwaZulu-Natal, South Africa. Until recently the conservation efforts targeting this species was hampered by lack of international recognition. Photograph by C.T. Downs, CC BY 4.0.
Dedicated researchers have recognised the importance of determining population size and raising the awareness of the plight of the Cape parrot and the forests for which it is a flagship species. Current abundance of the Cape parrot is relatively low but stable, with an estimate of fewer than 1,600 birds in the wild (Downs et al., 2014). Estimates are based on an annual census held since 1998, organized by citizen scientists. For the Cape parrot, tardy genetic recognition of full species status was overcome by conservationists’ perseverance. We must be vigilant if we want to protect other still-hidden species from future extinction.
3.4.3 Measuring species diversity
Biologists have developed three quantitative measures of species diversity as a means of measuring and comparing species diversity (Figure 3.7):
- Alpha diversity (or species richness), the most commonly referenced measure of species diversity, refers to the total number of species found in a particular biological community, such as a lake or a forest. Bwindi Forest in Uganda, with an estimated 350 bird species, has one of the highest alpha diversities of all African ecosystems.
- Gamma diversity describes the total number of species that occur across an entire region, such as a mountain range or continent, that includes many ecosystems. The Albertine Rift, which includes Bwindi Forest, has more than 1,074 species of birds, a very high gamma diversity for such a small region.
- Beta diversity connects alpha and gamma diversity. It describes the rate at which species composition changes across a region. For example, if every wetland in a region was inhabited by a similar suite of plant species, then the region would have low beta diversity; in contrast, if several wetlands in a region had plants communities that were distinct and had little overlap with one another, the region would have high beta diversity. Beta diversity is calculated as gamma diversity divided by alpha diversity. The beta diversity for forest birds of the Albertine Rift is about 3.0, if each ecosystem in the area has about the same number of species as Bwindi Forest.
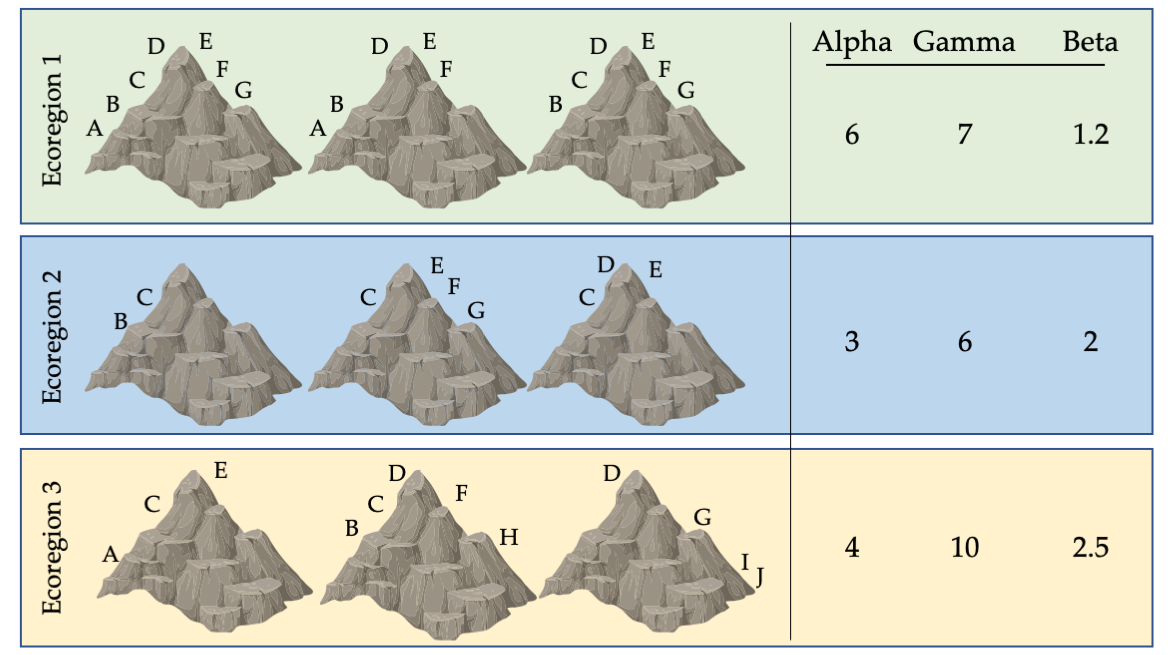
Figure 3.7 Biodiversity indices for nine mountain peaks across three ecoregions. Each symbol represents a different species; some species have populations on only one peak, while others are found on two or more peaks. The variation in species richness on each peak results in different alpha, gamma, and beta diversity values for each ecoregion. This variation has implications for how we divide limited resources to maximise protection. If only one ecoregion can be protected, ecoregion 3 may be a good choice because it has high gamma (total) diversity. However, if only one peak can be protected, should a peak in ecoregion 1 (with many widespread species) or ecoregion 3 (with several unique, range-restricted species) be protected? After Primack, 2012, CC BY 4.0.
It is important to note that alpha, beta, and gamma diversity describe only part of what is meant by biodiversity. For example, none of these three terms completely account for genetic diversity, which allows species to adapt as conditions change (Section 8.7.1). It also neglects the importance of ecosystem diversity, which results from the collective response of species to their dynamic environment. However, these diversity measures are useful for comparing different regions, and identifying locations with high concentrations of native species that should be protected.
3.4.4 How many species exist?
To date, taxonomists have described about 1.5 million species that share this planet with us (Costello et al., 2012). While this total may seem impressive, available evidence suggests that this estimate vastly underestimates the true extent of Earth’s biodiversity. In fact, even now, after all the exploration in years gone by, several thousand new species are being described each year. Many new discoveries are made by skilled researchers recognising new species by being able to discern variation in morphological characters; that includes the discoveries of a new small forest antelope from West Africa (Colyn et al., 2010) and a new species of shark off Mozambique (Ebert and Cailliet, 2011). Such discoveries can also be rather surprising and unexpected. For example, an amateur botanist recently discovered two new flowering plants in the heavily studied Cape Floristic Region (Bello et al., 2015). Similarly, the lesula (Cercopithecus lomamiensis)—a species of monkey long known to local hunters—was only formally described after biologists discovered this “different” monkey on a leash in a remote village of the DRC (Hart et al., 2012). Some recent discoveries even include entire new communities in unexpected places. For example, in 2007, grassland surveys by citizen scientists in an area starting 5 km from South Africa’s Johannesburg metropolitan area found previously unknown populations of five threatened bird species, as well as a number of regionally threatened birds and mammals; these discoveries were instrumental in recognising this area as the Devon Grasslands Important Birding Area (Marnewick et al., 2015).
New genetic technologies have highlighted that there are many thousands of species yet to be described.
The most exciting and newsworthy discoveries of new species generally involve higher-level taxa, especially living fossils. For example, in 1938, biologists across the world were stunned by the report of a strange fish caught in the Indian Ocean off South Africa. This fish, subsequently named coelacanth (Latimeria chalumnae, CR), belongs to a group of marine fishes that were common in ancient seas but were thought to have gone extinct 65 million years ago. Coelacanths are of interest to evolutionary biologists because they show certain features of muscles and bones in their fins that are comparable to the limbs of the first vertebrates that crawled onto land. Following the initial discovery, coelacanths have been found along Africa’s Indian Ocean coast from South Africa to the Comoros and through to Kenya. Unfortunately, the entire coelacanth population, estimated at fewer than 500 individuals, is currently highly threatened because of ongoing fishing pressures (Musick, 2000).
Although field surveys have proven to be of great importance for discovering new species and populations, perhaps the greatest taxonomic progress has come from advances in genetic analyses which help to separate cryptic species previously lumped under more widespread species. For example, advances in genetic research recently highlighted that the African clawed frog (Xenopus laevis, LC)—a popular model organism in biomedical research—consists of seven distinct species (Evans et al., 2015). Similarly, using new genetic methods, scientists recently confirmed that the slender-snouted crocodile (Mecistops cataphractus, CR) consists of two different species, one endemic to West Africa and the other to Central Africa (Shirley et al., 2018).
Estimates suggest there are somewhere between 1–6 billion distinct species on Earth. The most diverse group of species is bacteria.
The presence of so many undiscovered species and communities makes precise estimates of species diversity incredibly difficult, especially in Africa where so many areas remain scientifically unexplored. Our most recent estimates, combining genetic analysis of well-known groups with mathematical patterns, suggests there are between 1–6 billion distinct species on Earth (Table 3.1) of which there are only about 163 million animals and 340 thousand plants (Larsen et al., 2017)—this is obviously much greater than the current catalogue of 1.5 million species! Given the amount of new species that continue to hide in plain sight, so to speak, there is no doubt that a great number of species and communities are waiting to be discovered by eager African adventurers over the next several decades.
Table 3.1 Estimated living biomass and number of species for each kingdom of life, following the seven-kingdom system (Ruggiero et al., 2015). Note how plants weigh the most, but bacteria have the most species.
Kingdom |
Weight (Gt)a |
Number of species (in million) |
% of all speciesb |
Number of described speciesc |
% of described species |
Animals |
2 |
163 |
7 |
1,205,336 |
< 1 |
Fungi |
12 |
165 |
7 |
135,110 |
< 0.1 |
Plants |
450 |
0.382c |
< 0.5 |
364,009 |
95 |
Chromista |
Unknown |
0.025c |
< 0.5 |
23,428 |
94 |
Protozoans |
4 |
163 |
7 |
2,686 |
0.1 |
Archaea |
7 |
0.0005 |
< 0.5 |
377 |
75 |
Bacteria |
70 |
1,746 |
78 |
9,982 |
0.1 |
a As gigatonnes of carbon, from Bar-On et al., 2018
b From Larsen et al. (2017)’s Table 1, Scenario 1
c From http://www.catalogueoflife.org
3.4.5 Where are most species found?
Because it is so hard to obtain accurate estimates of species numbers, many conservation biologists have recently started to focus their efforts on understanding and planning around patterns of species diversity. This makes sense: regions with many species of one taxon tend to also have many species of other taxa, so protecting one diverse group of species will likely also protect many other species, even if those other species are not well understood. Consequently, many conservation biologists see the forests of the Congo Basin, Albertine Rift, and West Africa as critical conservation priorities because these areas hold Africa’s greatest species concentrations, particularly birds, mammals, and butterflies. But there are very important outliers. For example, due to factors that include the geology and soil characteristics, size and variability of the environment, historical circumstances, or climatic conditions, none of these tropical forest areas have as many plant species as the Cape Floristic Region of South Africa—an area of unparalleled importance for plant diversity. Species diversity relationships may also break down at the local scale; for example, amphibians are likely more diverse in wet, shady riverbeds, whereas reptiles may be more diverse in drier, open habitats even if only tens of metres of space separate the reptiles from a riverbed full of amphibians.
By examining all these patterns of species diversity across the world, biologists have discovered at least two general frameworks governing species richness. The first framework is that stable ecosystems usually have many species, while ecosystems that were subjected to more recent glaciation usually have fewer species. This observation explains why tropical ecosystems are generally considered the world’s most species-rich environments (Table 3.2). While tropical grasslands, wetlands, and other ecosystems all hold relatively high species diversity, species richness of tropical forests are particularly noteworthy; even though these areas occupy only about 7% of Earth’s land surface, they contain over half of the world’s species (Corlett and Primack, 2010). This is, in a large part, due to the relatively large global distribution of the tropical forests and the diversity of geological history between these areas of South and Central America, Africa, Asia, and Australia, which has resulted in unique assemblages of species that have evolved in isolation from each other.
Table 3.2 Number of endemic and native mammal species as a function of the environment, for a range of African countries.
Country |
Dominant ecoregion |
Area (× 1000 km2) |
Number of endemic mammals |
Number of native mammals |
Mammals per 1000 km2 |
Seychelles |
Oceanic island |
0.45 |
2 |
24 |
53.3 |
Cabo Verde |
Oceanic island |
4.03 |
0 |
29 |
7.2 |
Rwanda |
Montane forest |
26.8 |
1 |
189 |
7.05 |
Eq. Guinea |
Lowland forest |
28.1 |
3 |
184 |
6.55 |
Burundi |
Montane forest |
27.8 |
1 |
144 |
5.18 |
Sierra Leone |
Varied Forest |
71.7 |
0 |
197 |
2.75 |
Zimbabwe |
Savannah |
391 |
0 |
204 |
0.52 |
Zambia |
Savannah |
753 |
5 |
242 |
0.32 |
Namibia |
Desert |
825 |
3 |
206 |
0.25 |
South Africa |
Varied |
1,221 |
31 |
307 |
0.25 |
South Sudan |
Sahel |
644 |
1 |
151 |
0.24 |
DRC |
Varied |
2,345 |
26 |
438 |
0.19 |
Niger |
Sahel |
1,267 |
0 |
134 |
0.11 |
Source: IUCN, 2019.
Tropical forests are not the only species-rich tropical ecosystem. Tropical coral reefs, colonies of tiny aquatic invertebrates that form entire ecosystems (Figure 3.8), are the marine equivalent of tropical forests both in terms of species richness and complexity. These areas not only provide homes for corals, but also for huge numbers of fish, molluscs, and marine mammals that find shelter in these highly productive and sheltered ecosystems. In Africa, tropical coral reefs are most widespread and diverse in coastal East Africa, but unique tropical coral reef communities can also be found along Mozambique and South Africa’s north-eastern coast.
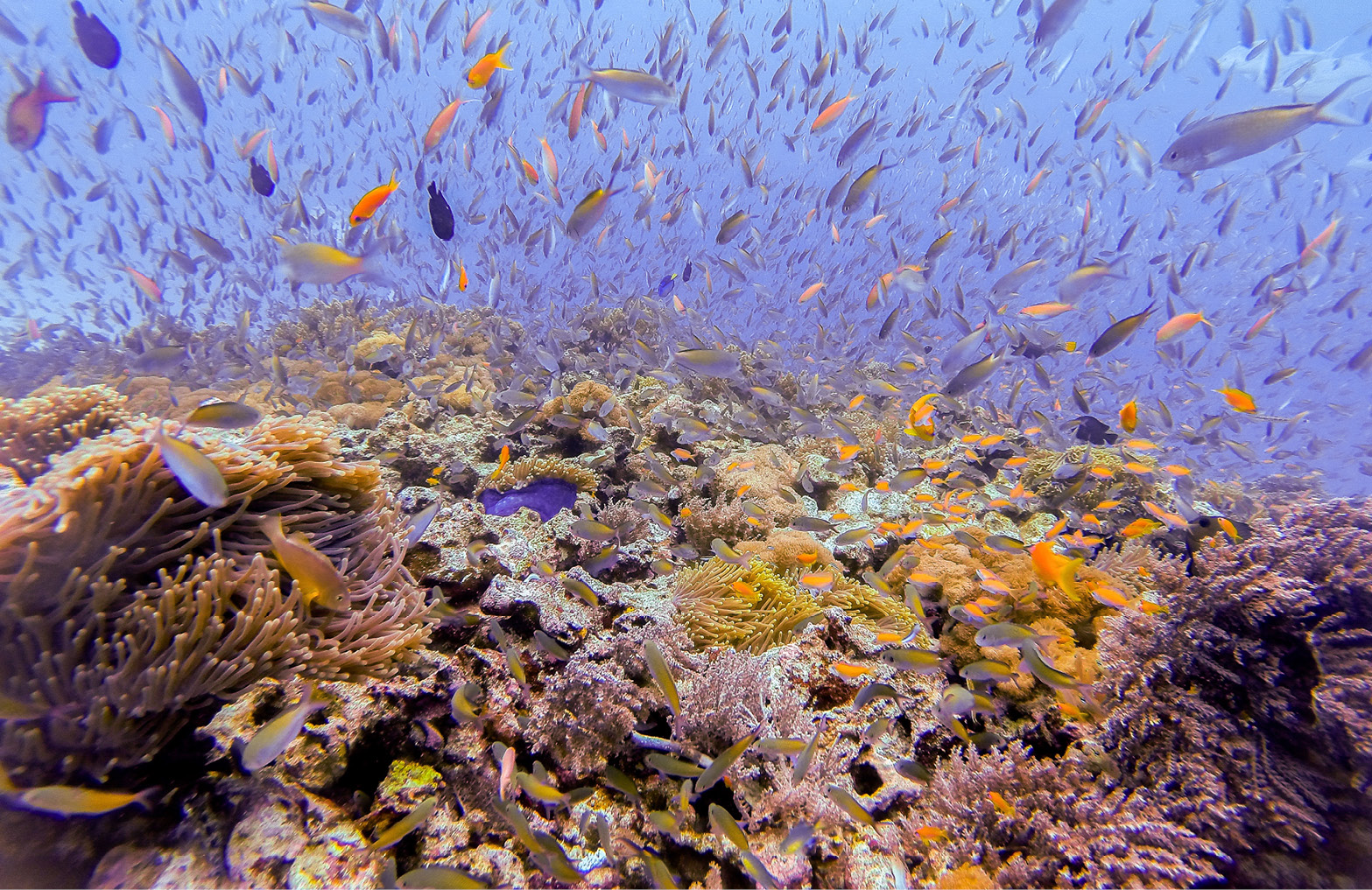
Figure 3.8 Coral reefs such as this one at Zanzibar’s Mnemba Atoll, off the north coast of Tanzania, are highly diverse underwater ecosystems composed of the accumulated skeletons of billions of tiny marine invertebrates. These underwater landscapes provide habitat for at least 25% of all marine species. Photograph by Kamal Karim, https://www.flickr.com/photos/118534047@N06/22449100152, CC BY 2.0.
High levels of species diversity, especially among plants, can also be found in ecosystems with a Mediterranean climate, such as southwestern Africa, as well as southwestern Australia, California, central Chile, and the Mediterranean Basin of southern Europe and North Africa. The climate of a Mediterranean-type ecosystem is characterised by cool, moist winters, hot, dry summers, resulting in distinctive plant adaptations such as short twigs and stiff leaves. A combination of special environmental factors, including a considerably old geological age, complex site characteristics (such as varied topography and soils), and frequent fires facilitated rapid speciation and helped to prevent any one species from dominating. Today, although regions with a Mediterranean climate cover only 2% of Earth’s surface, 20% of all plant species are found here (Underwood et al., 2009). The Cape Floristic Region—the only Mediterranean climate in Sub-Saharan Africa—is particularly important to conservationists as it has the highest concentration of higher plant diversity (over 9,000 species) in the world.
The second framework governing pattern of species diversity is that locations with high numbers of species usually hold many endemic species. The Cape Floristic Region, for example, boasts more than 6,200 endemic plant species, which include 12 endemic families and 160 endemic genera. Similarly, Lake Malawi holds nearly 14% of the world’s freshwater fishes (500–1,000 species, totals vary by source), with more than 90% of those being endemic.
Biogeographic transition zones—also known as ecotones—regions where different ecosystems meet and overlap, are a special case of areas that contain great species diversity and high levels of endemicity. These areas share environmental factors of two or more environments, allowing for the mixture of biodiversity from those component environments, while unique features within these areas often also give rise to unique species. A case in point is the Maputaland Centre of Endemism, situated in far southern Mozambique. Here, biological communities from northern tropical and southern temperate ecosystems overlap, resulting in surprisingly high levels of species richness as well as endemism (van Wyk, 1996).
Today is an exciting time of biological exploration. Methods and technologies for exploration are improving rapidly, and we are learning more about the value and function the diversity of life on Earth. As genetic techniques advance and become more accessible, an increasing number of people are participating in recording the presence of species in locations around the world; this includes amateur naturalists and citizen scientists who contribute to bird surveys, plant walks, and other natural history activities. With this increased knowledge of biodiversity also comes an acute awareness that human activities damage ecosystems and reduce diversity. Hopefully this broader awareness will spur more people to take responsibility to protect and restore that biodiversity.
3.5 Summary
- Earth’s biodiversity includes the entire range of living species (species diversity), the genetic variation that occurs among individuals within a species (genetic diversity), and, at a higher level, the biological communities in which species live and their associations with the physical and chemical environment (ecosystem diversity).
- For practical purposes, most ecologists and conservationists identify species in the field according to their morphology, although improvements in genetic techniques are allowing more species to be identified according to their evolutionary past, revealing many cryptic species that people did not realise were there.
- There are several ways to measure and compare biodiversity. The most popular measurement is species richness in a particular community, such as a forest or grassland (alpha diversity), species richness across a larger landscape, such as a mountain range (gamma diversity), and the rate of change of species composition as one crosses a large region (beta diversity).
- It is estimated that there may be as many as 2 billion species on Earth. Most species already described are insects, while the best-known species include birds and mammals. The majority of species still need to be discovered.
- Variation in climate, topography, and geological age are all factors that affect patterns of species richness. Geological age and complexity provide environmental variation, which in turn allows opportunities for genetic isolation, local adaptation, and speciation, given enough time. Tropical forests, coral reefs, and Mediterranean-type ecosystems host a disproportionately large amount of the world’s biodiversity.
3.6 Topics for Discussion
- Think of any group of species (birds, trees, or maybe insects) that can be found in the area where you live. Do you think it is important to be able to identify these species? Why? How many species can you personally identify at this moment? What steps would you take to learn to identify more species?
- Which ecosystems in your country are particularly species-rich, and which are species-poor? Describe some factors that make ecosystems species-rich or species-poor.
- Where in Africa do you think most undescribed species are lurking? Explain your answer.
3.7 Suggested Readings
Ceballos, G., and P.R. Ehrlich. 2009. Discoveries of new mammal species and their implications for conservation and ecosystem services. Proceedings of the National Academy of Sciences 106: 3841–46. https://doi.org/10.1073/pnas.0812419106 Even familiar taxa contain many undescribed species.
Bar-On, Y.M., R. Phillips, and R. Milo. 2018. The biomass distribution on Earth. Proceedings of the National Academy of Sciences 25: 6505–11. https://doi.org/10.1073/pnas.1711842115 Note the impact of humans on the composition of life on Earth.
Ebach, M.C., J.J. Morrone, L.R. Parenti, et al. 2007. International code of area nomenclature. Journal of Biogeography 35: 1153–57. https://doi.org/10.1111/j.1365-2699.2008.01920.x Scientists also grapple with confusing terminology when describing biodiversity.
Gippoliti, S., F.P.D. Cotterill, D. Zinner, et al. 2018. Impacts of taxonomic inertia for the conservation of African ungulate diversity: An overview. Biological Reviews 93: 115–30. https://doi.org/10.1111/brv.12335 Taxonomic inertia, or the delay in recognising distinct species, slows conservation efforts.
Hart, J.A., K.M. Detwiler, C.C. Gilbert, et al. 2012. Lesula: A new species of Cercopithecus monkey endemic to the Democratic Republic of Congo and implications for conservation of Congo’s Central Basin. PLoS ONE 7: e44271. https://doi.org/10.1371/journal.pone.0044271 Many species known to local people still need to be formally described.
Joppa, L.N., D.L. Roberts, and S.L. Pimm. 2011. The population ecology and social behavior of taxonomists. Trends in Ecology and Evolution 26: 551–53. https://doi.org/10.1016/j.tree.2011.07.010 The number of taxonomists and the number of species described per year are steadily increasing.
Laikre, L., F.W. Allendorf, L.C. Aroner, et al. 2010. Neglect of genetic diversity in implementation of the Convention on Biological Diversity. Conservation Biology 24: 86–88. https://doi.org/10.1111/j.1523-1739.2009.01425.x A greater emphasis on genetic diversity needs to be part of conservation efforts.
Larsen, B.B., E.C. Miller, M.K. Rhodes, et al. 2017. Inordinate fondness multiplied and redistributed: The number of species on Earth and the new pie of life. Quarterly Review of Biology 92: 229–65. https://doi.org/10.1086/693564 We have much to learn about life on Earth.
Minhós, T., E. Wallace, M.J.F. da Silva, et al. 2013. DNA identification of primate bushmeat from urban markets in Guinea-Bissau and its implications for conservation. Biological Conservation 167: 43–49. https://doi.org/10.1016/j.biocon.2013.07.018 Misidentifications could have significant conservation implications.
Bibliography
Bar-On, Y.M., R. Phillips, and R. Milo. 2018. The biomass distribution on Earth. Proceedings of the National Academy of Sciences 25: 6505–11. https://doi.org/10.1073/pnas.1711842115
Bello A., C.H. Stirton, S.B.M. Chimphango, et al. 2015. Psoralea diturnerae and P. vanberkelae (Psoraleeae, Fabaceae): Two new species restricted to the core Cape Region of South Africa. PhytoKeys 44: 97–107. https://doi.org/10.3897/phytokeys.44.8999
Benjamin-Fink, N., and B.K. Reilly. 2017. Conservation implications of wildlife translocations: The state’s ability to act as conservation units for wildebeest populations in South Africa. Global Ecology and Conservation 12: 46–58. https://doi.org/10.1016/j.gecco.2017.08.008
Bercovitch, F.B., P.S.M. Berry, A. Dagg, et al. 2017. How many species of giraffe are there? Current Biology 27: R136–R137. https://doi.org/10.1016/j.cub.2016.12.039
BirdLife International. 2017. Poicephalus robustus. The IUCN Red List of Threatened Species 2017: e.T119194858A119196714. http://doi.org/10.2305/IUCN.UK.2017-3.RLTS.T119194858A11919 6714.en
Blanc, J. 2008. Loxodonta africana. The IUCN Red List of Threatened Species 2008: e.T12392A3339343. http://doi.org/10.2305/IUCN.UK.2008.RLTS.T12392A3339343.en
Brown, D.M., R.A. Brenneman, K.P. Koepfli, et al. 2007. Extensive population genetic structure in the giraffe. BCM Biology 5: 57. https://doi.org/10.1186/1741-7007-5-57
CBD (Center for Biological Diversity). 2015. Petition to reclassify and uplist African elephants from Threatened to Endangered under the Endangered Species Act as two separate species: forest elephants (Loxodonta cyclotis) and savannah elephants (Loxodonta africana) (Tucson: CBD). https://www.biologicaldiversity.org/species/mammals/pdfs/African_Elephant_Uplisting_Petition.pdf
Ceballos, G., and P.R. Ehrlich. 2009. Discoveries of new mammal species and their implications for conservation and ecosystem services. Proceedings of the National Academy of Sciences 106: 3841–46. https://doi.org/10.1073/pnas.0812419106
Coetzer, W.R., C.T. Downs, M.R. Perrin, et al. 2015. Molecular systematics of the Cape Parrot (Poicephalus robustus): Implications for taxonomy and conservation. PLoS ONE 10: e0133376. https://doi.org/10.1371/journal.pone.0133376
Collinson, J.M., M. Päckert, Y. Lawrie, et al. 2018. Taxonomic status of the Liberian Greenbul Phyllastrephus leucolepis and the conservation importance of the Cavalla Forest, Liberia. Journal of Ornithology 159: 19–27. https://doi.org/10.1007/s10336-017-1477-0
Colyn, M., J. Hulselmans, G. Sonet, et al. 2010. Discovery of a new duiker species (Bovidae: Cephalophinae) from the Dahomey Gap, West Africa. Zootaxa 2637: 1–30. http://doi.org/10.11646/zootaxa.2637.1.1
Corlett, R., and R.B. Primack. 2010. Tropical Rainforests: An Ecological and Biogeographical Comparison (Malden: Wiley-Blackwell). https://doi.org/10.1002/9781444392296
Costello, M.J., S. Wilson, and B. Houlding. 2012. Predicting total global species richness using rates of species description and estimates of taxonomic effort. Systematic Biology 61: 871–83. http://doi.org/10.1093/sysbio/syr080
Davis A.P., T.W. Gole, S. Baena, et al. 2012. The impact of climate change on indigenous Arabica coffee (Coffea arabica): Predicting future trends and identifying priorities. PLoS ONE 7: e47981. http://doi.org/10.1371/journal.pone.0047981
de Jong, Y.A., and T.M. Butynski. 2010. Three sykes’s monkey Cercopithecus mitis × vervet monkey Chlorocebus pygerythrus hybrids in Kenya. Primate Conservation 25: 43–56. https://doi.org/10.1896/052.025.0109
Dijkstra, K.-D.B., J. Kipping, and M. Nicolas. 2015. Sixty new dragonfly and damselfly species from Africa (Odonata). Odonatologica 44: 447–678.
Downs, C.T., M. Pfeiffer, and L. Hart. 2014. Fifteen years of annual Cape Parrots (Poicephalus robustus) censuses: Current population trends and conservation contributions. Ostrich 85: 273–80. https://doi.org/10.2989/00306525.2014.959088
Ebert, D.A., and G.M. Cailliet. 2011. Pristiophorus nancyae, a new species of sawshark (Chondrichthyes: Pristiophoridae) from southern Africa. Bulletin of Marine Science 87: 501–12. https://doi.org/10.5343/bms.2010.1108
Evans B.J., T.F. Carter, E. Greenbaum, et al. 2015. Genetics, morphology, advertisement calls, and historical records distinguish six new polyploid species of African clawed frog (Xenopus, Pipidae) from West and Central Africa. PLoS ONE 10: e0142823. https://doi.org/10.1371/journal.pone.0142823
Fennessy, J., T. Bidon, F. Reuss, et al. 2016. Multi-locus analysis reveals four giraffe species instead of one. Current Biology 26: 2543–49. https://doi.org/10.1016/j.cub.2016.07.036
Gerlach, J. 2009. Pachnodus velutinus. The IUCN Red List of Threatened Species 2009: e.T40091A10304648. http://doi.org/10.2305/IUCN.UK.2009-2.RLTS.T40091A10304648.en
Goodwin, Z.A., D.J. Harris, D. Filer, et al. 2015. Widespread mistaken identity in tropical plant collections. Current Biology 25: R1066–R1067. https://doi.org/10.1016/j.cub.2015.10.002
Grobler, J.P., I. Rushworth, J.S. Brink, et al. 2011. Management of hybridization in an endemic species: decision making in the face of imperfect information in the case of the black wildebeest—Connochaetes gnou. European Journal of Wildlife Research 57: 997–1006. http://doi.org/10.1007/s10344-011-0567-1
Groves, C.P., F.P.D. Cotterill, S. Gippoliti, et al. 2017. Species definitions and conservation: A review and case studies from African mammals. Conservation Genetics 18: 1247–`156. https://doi.org/10.1007/s10592-017-0976-0
Hart, J.A., K.M. Detwiler, C.C. Gilbert, et al. 2012. Lesula: A new species of Cercopithecus monkey endemic to the Democratic Republic of Congo and implications for conservation of Congo’s Central Basin. PLoS ONE 7: e44271. http://doi.org/10.1371/journal.pone.0044271
IUCN, 2019. The IUCN Red List of Threatened Species. http://www.iucnredlist.org
Jackson, C.R., and M.P. Robertson. 2011. Predicting the potential distribution of an endangered cryptic subterranean mammal from few occurrence records. Journal for Nature Conservation 19: 87–94. http://doi.org/10.1016/j.jnc.2010.06.006
Larsen, B.B., E.C. Miller, M.K. Rhodes, et al. 2017. Inordinate fondness multiplied and redistributed: The number of species on Earth and the new pie of life. Quarterly Review of Biology 92: 229–65. https://doi.org/10.1086/693564
Maree S., N.C. Bennett, and G.N. Bronner. 2016. A conservation assessment of Neamblysomus julianae. In: The Red List of Mammals of South Africa, Swaziland and Lesotho, ed. by M.F. Child, et al. (Cape Town and Johannesburg: SANBI and EWT).
Maree S. 2017. Planning for persistence of a Juliana’s Golden Mole (Neamblysomus julliane) subpopulation threatened by urban development on the Bronberg Ridge of Pretoria (Tshwane), South Africa. IUCN/SSC Afrotheria Specialist Group Newsletter 13: 24–33. https://www.afrotheria.net/newsletter.php
Maree, S. 2015. Neamblysomus julianae. The IUCN Red List of Threatened Species 2015: e.T1089A21285354. http://doi.org/10.2305/IUCN.UK.2015-2.RLTS.T1089A21285354.en
Marnewick, M.D., E.R. Retief, N.T. Theron, et al. 2015. Important Bird and Biodiversity Areas of South Africa (Johannesburg: BirdLife South Africa). https://www.birdlife.org.za/images/IBA/Documents/IBA%20Version%202.pdf
Minhós, T., E. Wallace, M.J.F. da Silva, et al. 2013. DNA identification of primate bushmeat from urban markets in Guinea-Bissau and its implications for conservation. Biological Conservation 167: 43–49. https://doi.org/10.1016/j.biocon.2013.07.018
Mondol, S., I. Moltke, J. Hart, et al. 2015. New evidence for hybrid zones of forest and savanna elephants in Central and West Africa. Molecular Ecology 24: 6134–47. https://doi.org/10.1111/mec.13472
Musick, J.A. 2000. Latimeria chalumnae. The IUCN Red List of Threatened Species 2000: e.T11375A3274618. http://doi.org/10.2305/IUCN.UK.2000.RLTS.T11375A3274618.en
Mynhardt, S., S. Maree, I. Pelser, Net al. 2015. Phylogeography of a morphologically cryptic golden mole assemblage from South-Eastern Africa. PLoS ONE 10: e0144995. https://doi.org/10.1371/journal.pone.0144995
Nichols, E., T.A. Gardner, C.A. Peres, et al. 2009. Co‐declining mammals and dung beetles: An impending ecological cascade. Oikos 118: 481–87. https://doi.org/10.1111/j.1600-0706.2009.17268.x
Parker, J., A.J. Helmstetter, D. Devey, et al. 2017. Field-based species identification of closely-related plants using real-time nanopore sequencing. Scientific Reports 7: 8345. https://doi.org/10.1038/s41598-017-08461-5
Pennisi, E. 2016. Pocket DNA sequencers make real-time diagnostics a reality. Science 351: 800–01. https://doi.org/10.1126/science.351.6275.800
Plumptre, A.J., and T. Wronski. 2013. Tragelaphus scriptus Bushbuck. In: Mammals of Africa: v. VI: Pigs, Hippopotamuses, Chevrotain, Giraffes, Deer and Bovids, ed. by J. Kingdon and M. Hoffmann (London: Bloomsbury Publishing).
Primack, R.B. 2012. A Primer for Conservation Biology (Sunderland:Sinauer).
Ruggiero, M.A., D.P. Gordon, T.M. Orrell, et al. 2015. A higher-level classification of all living organisms. PLoS ONE 10: e0119248. http://doi.org/10.1371/journal.pone.0119248
Salzburger, W., S. Baric, and C. Sturmbauer. 2002. Speciation via introgressive hybridization in East African cichlids? Molecular Ecology 11: 619–25. http://doi.org/10.1046/j.0962-1083.2001.01438.x
Shirley, M.H., A.N. Carr, J.H. Nestler, et al. 2018. Systematic revision of the living African slender-snouted crocodiles (Mecistops Gray, 1844). Zootaxa 4504: 151–93. http://doi.org/10.11646/zootaxa.4504.2.1
Takahara, T., T. Minamoto, and H. Doi. 2013. Using environmental DNA to estimate the distribution of an invasive fish species in ponds. PLoS ONE 8: e56584. https://doi.org/10.1371/journal.pone.0056584
Taylor, W.A., S. Mynhardt, and S. Maree. 2018. Family Chrysochloridae (Golden Moles). In: The Handbook of the Mammals of the World, v. 8, ed. by. D.E. Wilson, and R.A. Mittermeier (Barcelona: Lynx Ediciones).
Thomsen, P.F., J. Kielgast, L.L. Iversen, et al. 2012. Monitoring endangered freshwater biodiversity using environmental DNA. Molecular Ecology 21: 2565–73. https://doi.org/10.1111/j.1365-294X.2011.05418.x
Underwood, E.C., J.H. Viers, K.R. Klausmeyer, et al. 2009. Threats and biodiversity in the Mediterranean biome. Diversity and Distributions 15: 188–97. https://doi.org/10.1111/j.1472-4642.2008.00518.x
van Wyk, A.E. 1996. Biodiversity of the Maputaland Centre. In: The Biodiversity of African Plants, ed. by L.J.G. van der Maesen et al. (Dordrecht: Kluwer Academic).
van Wyk, A.M., D.L. Dalton, S. Hoban, et al. 2017. Quantitative evaluation of hybridization and the impact on biodiversity conservation. Ecology and Evolution 7: 320–30. http://doi.org/10.1002/ece3.2595
vaz Pinto, P., P. Beja, N. Ferrand, et al. 2016. Hybridization following population collapse in a critically endangered antelope. Scientific Reports 6: 18788. https://doi.org/10.1038/srep18788
Whittaker, R.H. 1975. Communities and Ecosystems (New York: Macmillan).
Willerslev, E., A.J. Hansen, J. Binladen, et al. 2003. Diverse plant and animal genetic records from holocene and pleistocene sediments. Science 300: 791–95. https://doi.org/10.1126/science.1084114
Wirminghaus, J.O., C.T. Downs, M.R. Perrin, et al. 2002. Taxonomic relationships of the subspecies of the Cape Parrot Poicephalus robustus (Gmelin). Journal of Natural History 36: 361–78. https://doi.org/10.1080/00222930010004250