6. Our Warming World
© 2019 J.W. Wilson and R.B. Primack, CC BY 4.0 https://doi.org/10.11647/OBP.0177.06
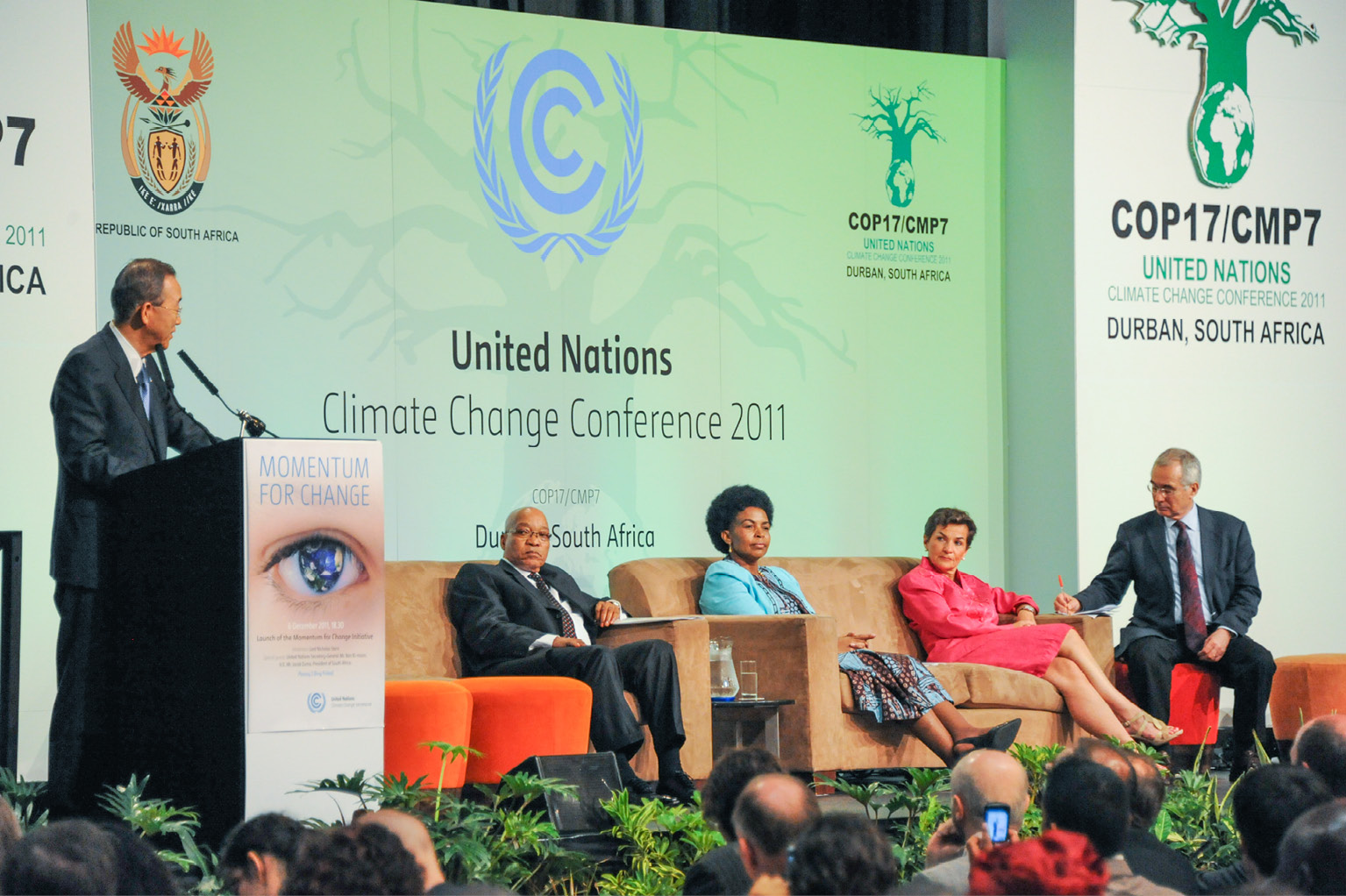
UN secretary general Ban Ki-moon introduces the Momentum for Change initiative at the UN Climate Change Conference, also known as COP17, held in Durban, South Africa in 2011. Photograph by UNFCCC, https://www.flickr.com/photos/unfccc/6470741719, CC BY 2.0.
Life-threatening heatwaves, drowning coastal towns, tens of thousands of displaced refugees… These words may very well describe a scene from the latest horror movie. But they also describe the nightmare scenario facing us humans in just a few decades if we continue to leave the threat of climate change under-addressed. This term, climate change (which is shorthand for anthropogenic climate change), refers to the complete set of climate characteristics—temperature; precipitation; pressure systems; wind patterns; and oceanic currents—that are changing both locally and regionally due to human influences. It is closely related to global warming, also called global heating, which describes the general trend of increasing global temperatures we see under climate change.
Climate change has the potential to render Earth unrecognisable from what any human has ever experienced. These changes will have an immense impact on ecosystem services, global economies, and our own quality of life. Yet, while there is much talk about these risks, there is too little action addressing its main causes. Some of the lack of action may be attributed to “climate change” and “future” often being used in the same sentence, giving politicians and industries a false impression that we can deal with climate change once we achieved sufficient economic growth. The reality could however not be further from the truth, as we already see signs of the changes to come here today (Table 6.1), including near-annual crop-failures, record-high temperatures, and record-strength coastal storms.
Table 6.1 Some examples illustrating how climate change is already impacting Africa.
Impact |
Evidence |
Increased temperatures and incidence of heat waves |
Global temperatures in 2016 were the warmest since modern recordkeeping began in 1880; the two previous records were set in 2015 and 2014 (Gillis, 2017). Heat waves are also hotter, longer, and over a larger area than before (Russo et al., 2016). |
Widespread droughts |
East Africa saw its worst drought in 60 years from mid-2011 to mid-2012. Over 250,000 people died; nearly 10 million more needed humanitarian assistance (Maxwell et al., 2014). The increased intensity of similar droughts in 2016 are directly attributable to climate change (Uhe et al., 2017). |
Rising sea levels |
Coastal floods disrupt lives and local economies in Ghana, Nigeria, and Benin almost every year. Coastal erosion has damaged commercial properties in The Gambia and Senegal, while the coastline retreated 35 m in some areas of Togo (Fagotto and Gattoni, 2016). |
Earlier spring activity |
Bloom dates for several plants, including commercially grown apple and pear trees in South Africa, are now between 1.6 and 4.2 days earlier per decade than 35 years ago (Grab and Craparo, 2011). |
Shifts in species ranges |
Malaria recently appeared in the highlands of Ethiopia, Kenya, Rwanda, and Burundi, in areas where it did not occur before (Siraj et al., 2014). |
Wildlife population declines |
Reporting rates for some bird species endemic to the Cape Floristic region declined by over 30% over the past 15 years (Milne et al., 2015). |
Thankfully, with the increase in understanding that our activities are creating a global crisis of epic proportions, the impacts of climate change are now being actively debated in the corridors of governments and major corporations. Politicians, the media, and others are also increasingly replacing “climate change” with more vivid language, like “climate crisis” and “climate emergency” (e.g. Carrington, 2019). This will hopefully encourage even more governments and industries to come to the table and cooperate like never before to address the fundamental drivers of climate change. Solving this global crisis requires an international multi-pronged approach that should include ecosystem protection and restoration (Chapter 10), direct species management (Chapter 11), and legislative action (Chapter 12). But before we consider the solutions, we will first investigate why climate change is happening, and how it will impact biodiversity over the coming decades.
6.1 Drivers of Climate Change
Greenhouse gases are essential for life on Earth. But too much of them cause Earth to heat up too much too fast, leading to climate change.
The climate change we are experiencing today is driven by human activities that increase greenhouse gas concentrations in Earth’s atmosphere. Although we mainly hear about greenhouse gases in the context of their contribution to climate change, they are in fact essential for life on Earth. Consider for a moment carbon dioxide’s (CO2) critical role in photosynthesis, and water vapour’s role in the formation of rain. Both of these gases are greenhouses gases. Greenhouse gases earn their name because they function much like the glass covering a greenhouse; they allow sunlight to easily pass through the atmosphere but trap the reflected heat energy so that it stays close to Earth’s surface. This greenhouse effect allows all the organisms on Earth, even us humans, to flourish. Without greenhouse gases, temperatures would drop, and our planet would be too cold to sustain life. However, high concentrations of greenhouse gases can also be harmful. Think for a moment of greenhouse gases as “blankets” covering the Earth’s surface: more “blankets” will trap more heat, giving rise to higher temperatures. This is exactly what is happening today—human activities are currently increased greenhouse gas concentrations in the atmosphere so much, and at such a fast pace, that Earth is heating up too fast for biodiversity to adapt to the changes.
Africa’s biggest contribution to climate change comes from the destruction of complex ecosystems, which leads to the loss of important carbon sinks.
At present, the single biggest cause of increased greenhouse gas concentrations is the burning of fossil fuels. Since the Industrial Revolution about 200–250 years ago, humans have become heavily dependent on the energy captured in these fuels—coal, oil, and natural gas—for activities such as transportation, heating, manufacturing, and electricity generation. Fossil fuels contain a high percentage of carbon, so when it is burned, that carbon is released into the atmosphere, generally as CO2. Consequently, since human populations started exploding and have been using fossil fuels at increased rates, the greenhouse effect has been significantly amplified.
While fossil fuel burning is currently the biggest overall driver of climate change, the greatest contribution from Africa is the destruction of carbon sinks, such as tropical forests (Box 6.1) and peatlands. Destroying these ecosystems contributes to rising atmospheric CO2 concentrations directly through burning of vegetation that releases carbon, and indirectly through the loss of vegetation that would otherwise extract CO2 from the atmosphere if they were still alive. The contribution of ecosystem loss to climate change is substantial: 13% of today’s global carbon emissions can be accounted for by tropical deforestation (IPCC, 2014). This impact is much stronger in Africa where deforestation accounts for 35% of the region’s overall climate change impacts (WRI, 2019). In comparison, Africa’s energy and agricultural sectors contribute 30% and 24%, respectively.
Box 6.1 Does Oil Palm Agriculture Threaten Biological Diversity in Equatorial Africa?
Acadia National Park, US National Park Service,
Bar Harbor, ME, USA.
Oil palm (Elaeis guineensis, LC) is among the fastest expanding crops in the world. Native to West Africa, this species produces more oil per hectare than any other cultivated crop in the world. It should thus come as no surprise that it has become world’s most popular source of vegetable oil. Tropical Africa is poised as a hotspot for new oil palm plantations (Linder, 2013; Vijay et al., 2016). Is this a good thing? Will the benefits from jobs and carbon sequestration outweigh the loss of native ecosystems?
To many people, oil palm cultivation presents a win-win situation. The industry provides jobs and economic stimulus (Figure 6.A) and claims that oil palms sequester carbon from the atmosphere (Burton et al., 2017). This could potentially help countries offset carbon emissions; they may even receive funding from carbon markets. Palm oil can also be used for cheap bioenergy production, and as an ingredient in food and household products (e.g. cooking oil, baked goods, salad dressings, shampoo, and soap). Consequently, demand is rapidly growing as sales of processed and packaged foods (today about 50% of packaged foods include palm oil as an ingredient) expand globally.
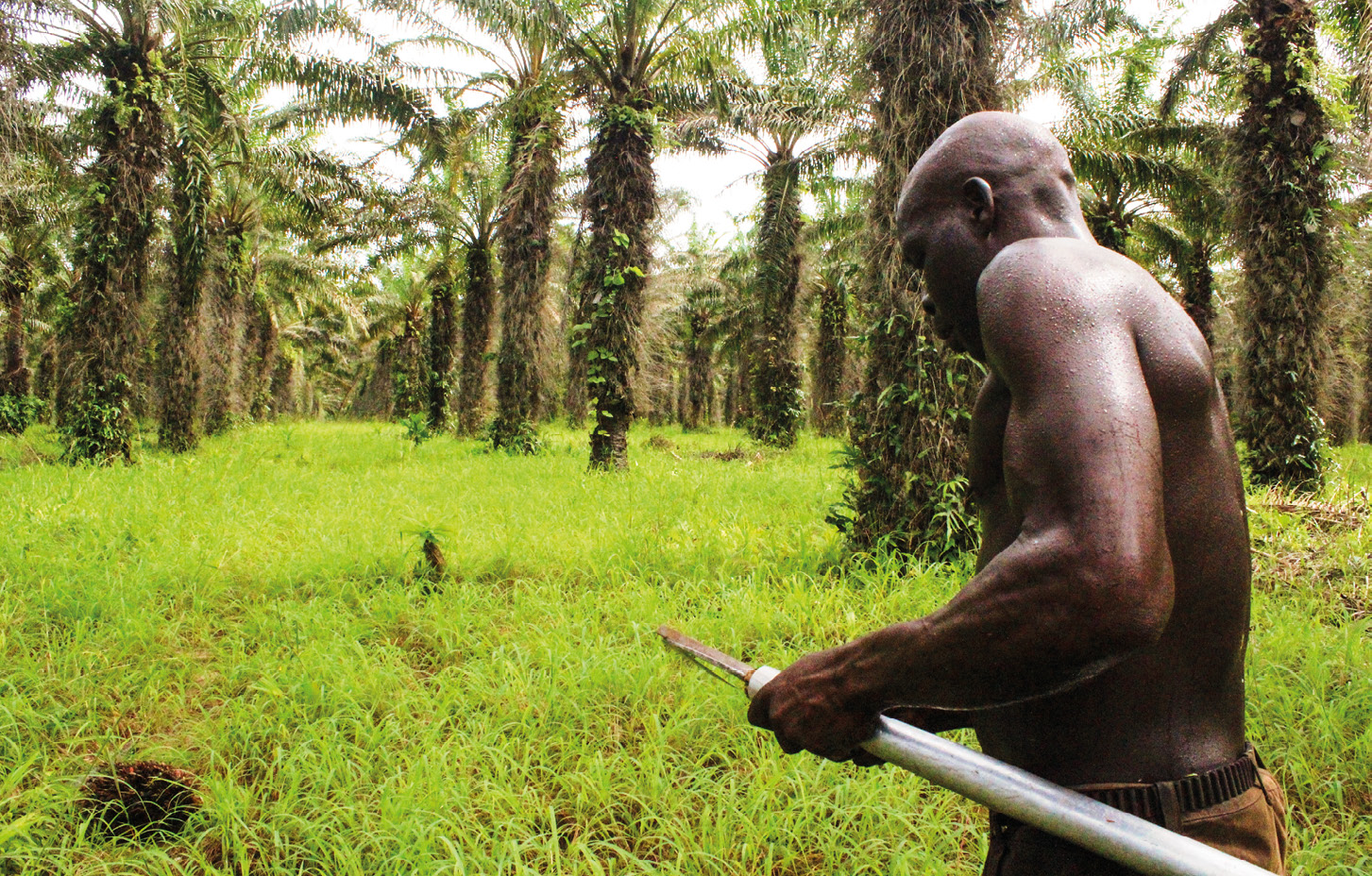
Figure 6.A A plantation worker getting ready to harvest oil palm fruit, locally known as red gold, in Côte d’Ivoire. Often associated with land grabbing, deforestation, biodiversity losses, and exploitation of local communities, there is a need for the palm oil industry to become more sustainable to provide lasting and meaningful benefits to local economies. Photograph by Donatien Kangah, https://commons.wikimedia.org/wiki/File:R%C3%A9colteur_de_r%C3%A9gimes_de_palme_1.jpg, CC BY-SA 4.0.
Oil palm plantations, however, are rarely developed in environmentally friendly ways that allow them to realise their potential value. Rather, it generally comes at a great ecological cost. For example, to ensure net positive carbon sequestration, oil palm plantations must be developed on degraded landscapes, rather than displacing intact ecosystems that are already very effective at sequestering carbon (Burton et al., 2017). In practice however, intact forests are more often logged to make space (and additional revenue) for oil palm plantations (Ordway et al., 2019), resulting in habitat loss and net positive carbon emissions. Oil palm plantations are also often associated with great societal costs, like land grabbing, exploitation of local people, and displacement of traditional activities (Linder and Palkovitz, 2016). The influx of migrant plantation workers puts further strain on the environment through unsustainable hunting of bushmeat. One study found that primate population sizes declined by 25–100% after palm plantation development in Côte d’Ivoire (Gonedelé et al., 2012).
Recently, Herakles Farms/SG Sustainable Oils, an American agribusiness company, attempted to develop a 730 km2 oil palm plantation in Cameroon. This land grab would have been one of the largest palm oil projects in Africa, nestled deep within Cameroon’s lowland tropical forests, one of the continent’s most biologically diverse and threatened ecosystems. The forests threatened by this development is situated adjacent to four protected areas that include two national parks (Linder and Palkovitz, 2016), and host 14 species of threatened primates, including the Nigeria-Cameroon chimpanzee (Pan troglodytes ellioti, EN) (Linder, 2013). Residents and environmental groups opposed the plantation because of possibly illegal activities by the company, the ecological consequences of the project, and because the local people would have received little, if any, benefit from the project. After protracted debate and struggle, including intimidation and the arrest of local social and environmental activists, the company withdrew its Cameroonian plans in 2013.
It seems that there is potential for oil palm plantations to be good for economic development, job creation, and conservation. But in practice, companies establishing these plantations frequently exploit local people and degrade local ecosystems. They sometimes even do this under the auspices of sustainability, arguing that low-impact activities by traditional peoples indicate that the area is already degraded and thus suitable for development. Hopefully, one day we can live in a world where palm oil companies and robust legal systems truly consider the protection of biodiversity and the rights of local people in those operations.
The link between human-induced climate change and atmospheric CO2 concentrations was first highlighted in the late 19th century (Arrhenius, 1896). However, it was not until the mid-1950s (e.g. Kaempffert, 1956) that scientists started to raise concerns about increasing CO2 concentrations in the atmosphere. By the 1980s, as global annual mean temperatures started to rise, consensus about climate change linked to CO2 began to spread among the broader public. Yet concrete steps to curb CO2 emissions would only be initiated decades later (Section 12.2.1). In the meantime, CO2 emissions continue to accelerate (Figure 6.1): more than 37 billion tonnes of carbon, a new record, were released into the atmosphere in 2018 (Jackson et al., 2018; Le Quéré et al., 2018). To put it in another way, during 2018, humans released on average over 100 million tonnes of CO2 into the atmosphere every day.
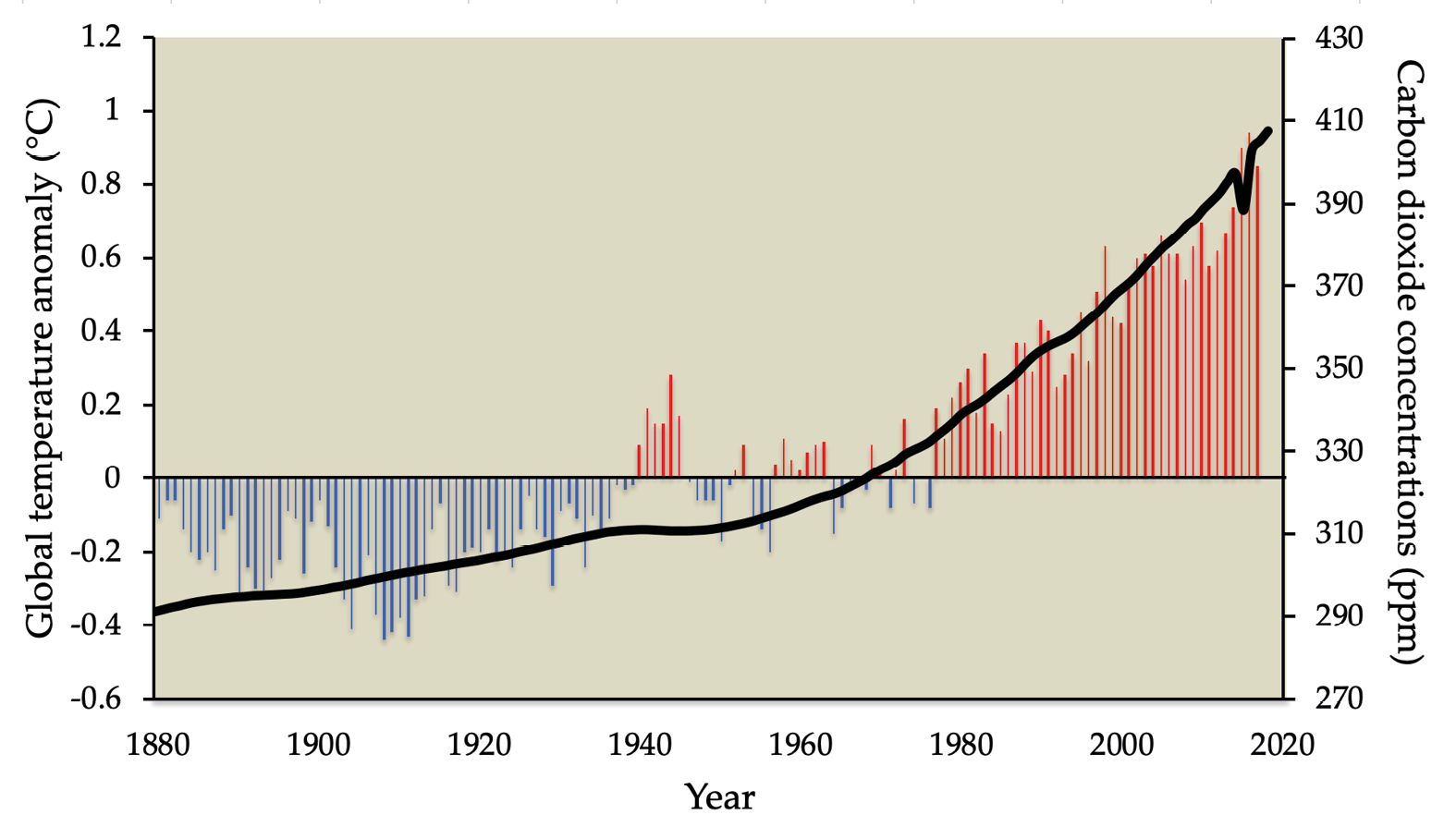
Figure 6.1 Human activities, notably the burning of fossil fuels and deforestation, have drastically increased atmospheric CO2 concentrations over the past century. As a result, average annual global temperatures are now much higher than they have been in the past. Temperatures are reported in terms of difference (anomaly) from average annual temperature from 1910–2000. Source for climate data: NOAA, 2018a. Sources for CO2 data: NASA, 2018 (before 2006); NOAA, 2018b (after 2005), CC BY 4.0.
The second-most important greenhouse gas that drives climate change is methane (CH4). Methane is a natural by-product emitted from decaying organic matter, most notably from wetlands that inhibit the speed of decomposition. These important ecosystem processes release methane into the atmosphere, albeit in relatively low concentrations. However, human activities have boosted methane emissions significantly over the past few centuries, through wasted food decaying at landfills, leaks from natural gas wells, an increase of industrial-scale cattle and dairy farms, and large-scale destruction of swamps and peatlands. Warmer temperatures also result in the drying of wetlands and peatlands; this drying speed up decomposition of organic material, which increases the rate of methane release. Methane currently constitutes 16% of all global greenhouse gas emissions released by humans (IPCC, 2014). This may not seem to be a major contribution; however, methane is 72 times more effective than CO2 in trapping radiation over a 20-year period (Forster et al., 2007), so even small increases in atmospheric methane can have dramatic effects.
The third important greenhouse gas that drives climate change is nitrous oxide (N2O), also known as laughing gas. Nitrous oxide is a by-product of synthetic fertilisers used in agriculture, burning of fossil fuels, and several industrial processes, and accounts for 6% of all human-caused greenhouse emissions (IPCC, 2014). However, it is even more potent than methane, and stays in the atmosphere for about 114 years, so the impact of one tonne of N2O is equivalent to 310 tonnes of CO2 over 100 years (Forster et al., 2007).
6.2 Predicting Earth’s Future Climate
Climate change forecasting is famously complex, with a great amount of uncertainty attached to the task. Most of us have been exposed to short-term (i.e. day-to-day) weather forecasts on television, radio, and newspapers. These daily weather forecasts are derived from current weather measurements while considering the historical record of past events that occurred during similar conditions. Some daily forecasts may also be created for as many as two weeks into the future, but these future outlooks are generally much less detailed. In contrast, forecasting climate change involves predicting novel weather conditions for several decades into the future. The general circulation models (GCM) used for climate change forecasting (Figure 6.2) also need to account for a great number of highly variable components, each affecting one another across the only planet we can adequately measure or examine (we have no other planet where we can test predictions). Among thousands of considerations, climatologists (scientists who study climate) need to account for how human activities might change over time, and how these activities will change the atmosphere’s composition. They also need to account for how much CO2 the world’s oceans and plants will absorb, and how wind and fire might influence these processes. Combining all the component parts, climatologists then need to estimate how increased temperatures will affect the polar ice caps, how the melting ice will affect oceanic conditions and currents which, in turn, will affect terrestrial conditions and weather patterns. Uncertainty also exists over interactive effects of some drivers. For example, higher temperatures increase evaporation and cloud cover which, in turn, will have a cooling effect (a similar short-term cooling effect, caused by an albedo effect, is noted after an ecosystem is cleared due to the bare ground’s ability to reflect more sunlight than it absorbs, Section 4.2.3). Because of the complexity of these and other variables going into climate models, a great number of research groups are encouraged to develop their own climate forecasts, each using a range of different scenarios on how human activity might change in the future.
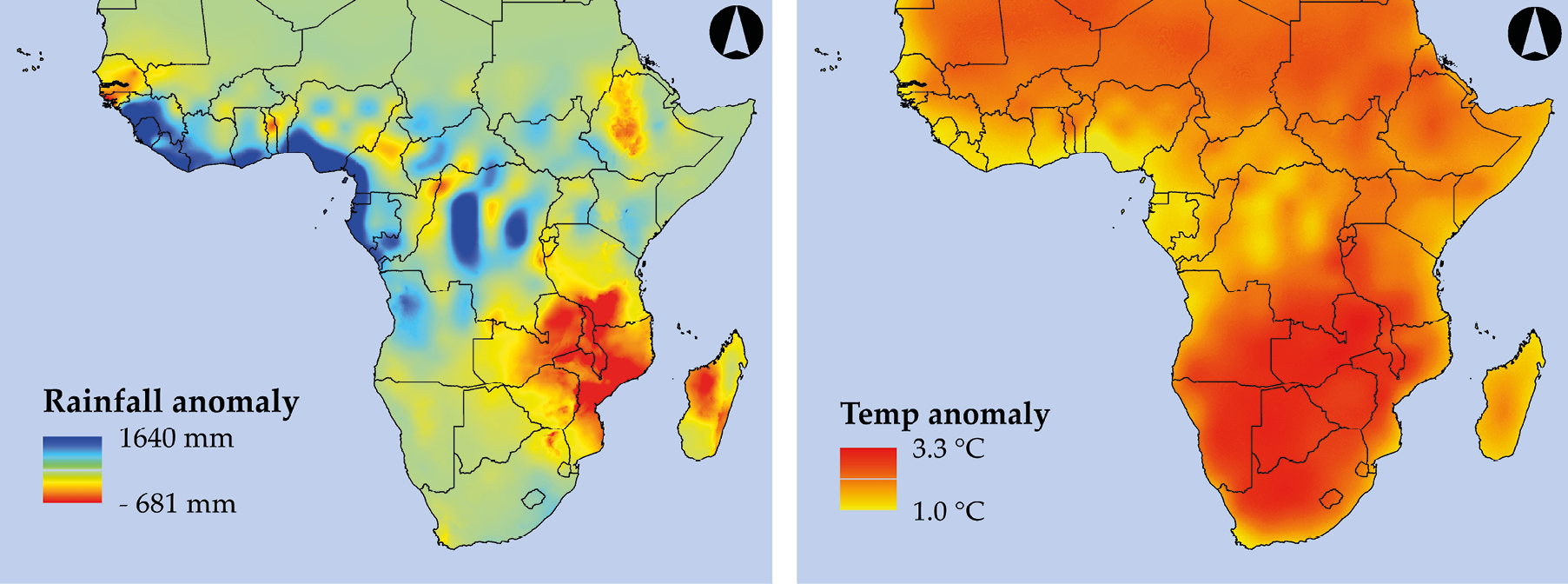
Figure 6.2 (Left) Annual precipitation (mm) and (Right) annual mean temperature (°C) shift predicted for Sub-Saharan Africa in 2070, assuming greenhouse gas emissions peak around 2080. Values presented as the amount of deviation from 1960-1990 averages. Some coastal areas of West and Central Africa are predicted of have more rain, but large areas of southeast Africa will get much drier. All of Africa is predicted to get hotter, with the greatest increases in southern Africa. Source: https://www.worldclim.org; model: GISS-E2-R. Map by Johnny Wilson, CC BY 4.0.
To further improve upon climate change forecasting, in 1988, the UN appointed a group of leading scientists, collectively known as the Intergovernmental Panel on Climate Change (IPCC), to study the implications of climate change. By regularly doing extensive reviews of all the evidence and climate science literature, the IPCC has found that, despite the complexity of climate models, results of all the models taken together exhibited significant agreement with changes already observed. Climate change models have also proven reliable in predicting responses of biodiversity to climate change (Fordham et al., 2018). Thus, while some fringe groups may continue to deny the validity of climate science, there is broad consensus among the world’s scientists that increased atmospheric greenhouse gases—caused by human activities—are causing the world’s climate to change, and it will continue to change in coming decades. While climatologists continue to improve on the finer details of their models, conservation biologists can and should confidently use the climate forecasts available for general conservation planning purposes.
Assuming human activities continue business as usual, and current greenhouse gas emission rates continue unabated, climatologists predict that average annual temperatures in Sub-Saharan Africa will increase by 0.5°C by 2050, compared to temperatures late in the 20th century (Serdeczny et al., 2017). The increase could be even greater, towards 4°C, if humans emit more greenhouse gases than predicted and Earth’s carbon storage systems underperform. Conversely, temperatures could warm less or more slowly if we manage to slow greenhouse gases emissions and better protect natural carbon sinks. Unfortunately, current evidence suggests that the higher temperature estimates seem more likely. For example, 2016 was the hottest year (since modern record-keeping) globally for the third straight year (Gillis, 2017) with temperatures already 0.9°C above late 20th century averages. Another climate record was set in April 2018, which was Earth’s 400th straight warmer-than-average month (NOAA, 2018c). Also, more locally, scientists observed that temperatures in some South African national parks reached temperature increases predicted for 2035 already in 2015 (van Wilgen et al., 2016).
6.3 The Impact of Climate Change
Climate change is not a new phenomenon. During the past 2 million years, there have been at least 10 cycles of global warming and cooling. When the polar ice caps melted during warming periods, sea levels rose to well above their earlier levels, and a larger portion of Earth experienced tropical climates. During cooling periods, the polar ice caps expanded, sea levels dropped, and tropical species’ ranges contracted. Sometimes these changes occurred gradually, which enabled the affected species to adapt. But the onset of some climate change periods was abrupt, causing major ecosystem disruptions and global mass extinction events (Section 8.1). Yet, nature recovered every time; many of the species we see today are survivors of previous climate change events. It is thus fair to ask why today’s climate change is of such concern to us.
6.3.1 Climate change’s impact on people
History provides us with many lessons to illustrate the impact of climate change on human societies. These lessons start with the earliest well-documented example of a societal collapse—that of the Middle East’s Natufian communities roughly 10,000 years ago—which has been attributed to climatic changes (Weiss and Bradley, 2001). Since then, climate change has regularly contributed to the collapse of complex human societies across the world. Notable examples of such collapses include the Akkadian Empire (the world’s first empire) of the Middle East (Carolin et al., 2019), Egypt’s Old Kingdom (who constructed the pyramids), Central America’s Classic Mayan civilisation, the USA’s first English colony (deMenocal, 2001), several Chinese dynasties (Wang et al., 2010), and the Late Bronze Age societies along the Mediterranean Sea (Kaniewski et al., 2013). Also, in Southern Africa, the fall of the Mapungubwe Kingdom has been attributed to crop failures and declining grazing lands due to regional droughts and warming cycles (O’Connor and Kiker, 2004).
Unlike the unavoidable natural climatic shifts that led to the historical societal collapses discussed above, we have brought today’s climatic change impacts upon ourselves. Because of our general lack of response in addressing the drivers of climate change, thousands of people will suffer the consequences. Prominently, many parts of Africa are already seeing higher temperatures and longer droughts (Engelbrecht et al., 2009). These conditions are compromising our quality of life (Watts et al., 2017) by leading to more intense wildfires (Jolly et al., 2015; Strydom and Savage, 2016), increased incidences of malaria (Siraj et al., 2014), increased crop failures (Myers et al., 2014; Medek et al., 2017), and increased competition for water (Flörke et al., 2018). Many coastal areas are also seeing storms increasing in intensity and frequency, exposing people living near large rivers, deltas, and estuaries to more frequent flooding (Figure 6.3) and storm surges (Fitchett and Grab, 2014). Sea level rise is expected to leave many low-lying oceanic islands uninhabitable within a few decades (Storlazzi et al., 2018). With all these impacts expected to increase the competition for space under an increasing human population, it would be wise for the world’s governments to start preparing for thousands of climate refugees that would need to be relocated in the near future (Merone and Tait, 2018).
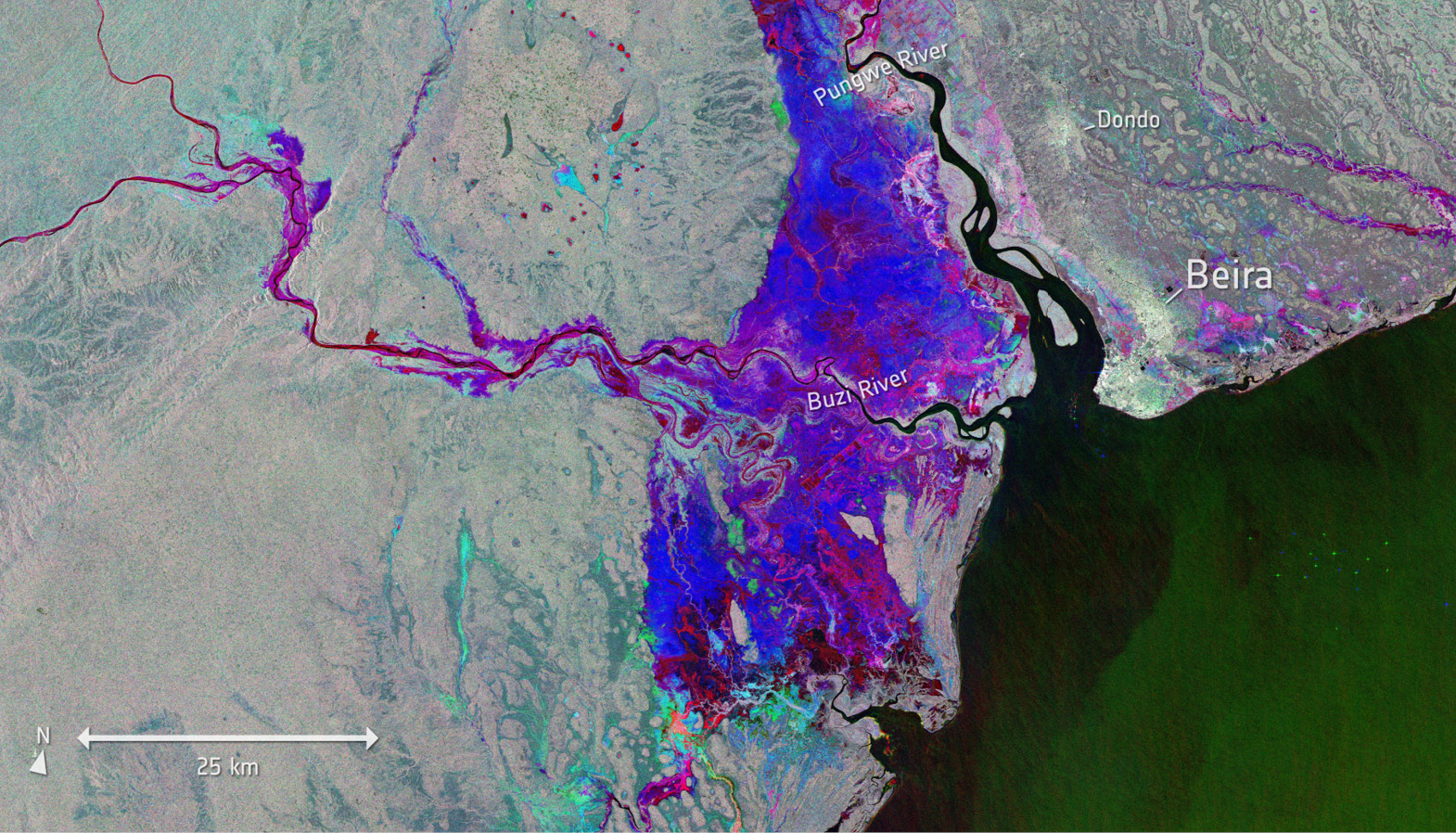
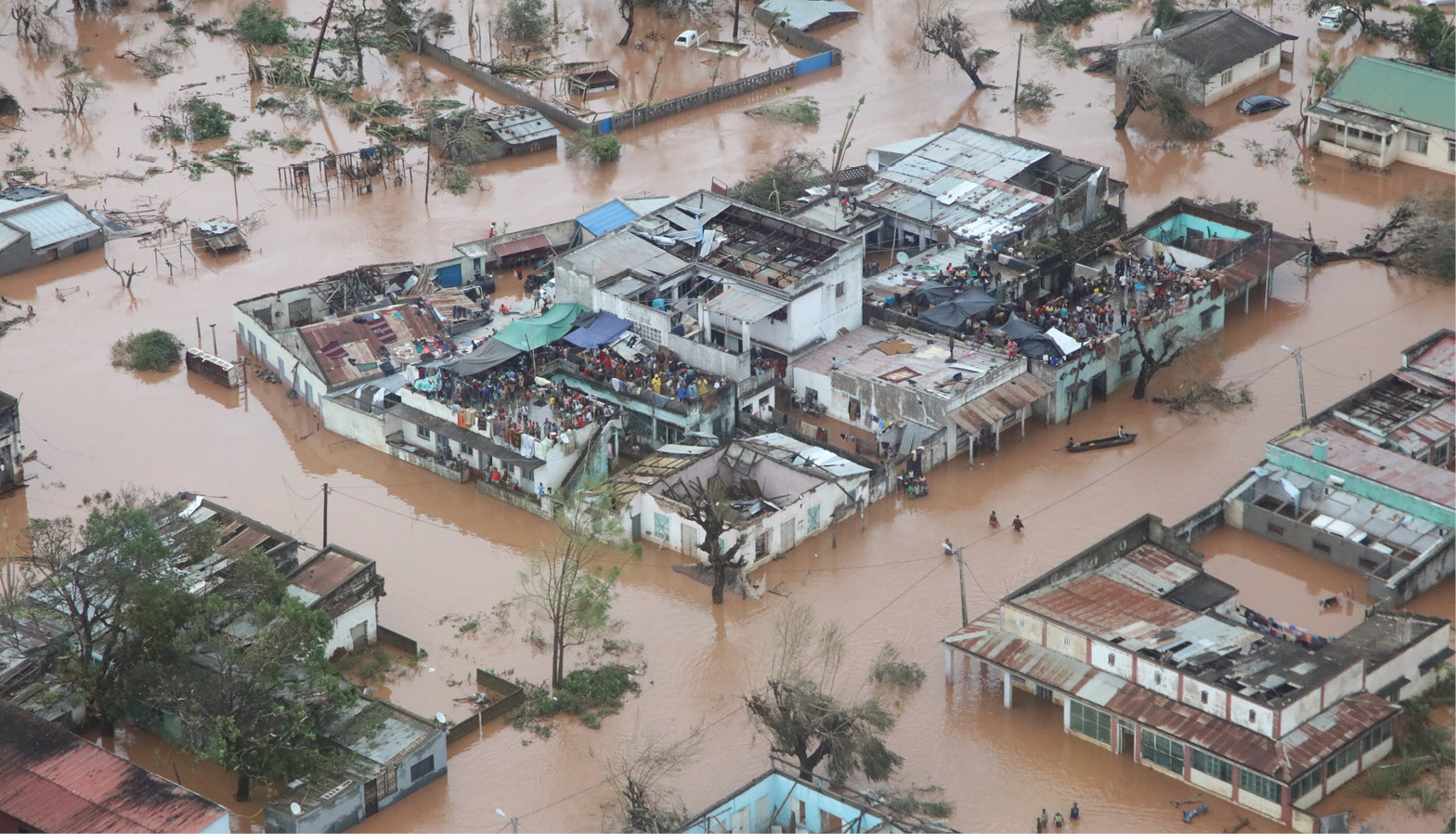
Figure 6.3 (Top) A Copernicus Sentinel-1 satellite image showing the extent of flooding (areas shown in blue) in central Mozambique after Cyclone Idai made landfall on 15 March, 2019. Photograph by European Space Agency, https://www.flickr.com/photos/europeanspaceagency/47477652401, CC BY-SA 2.0. (Bottom) People in Beira, Mozambique, taking refuge on rooftops to escape flooding brought by Cyclone Idai. Photograph by World Vision, https://www.flickr.com/photos/dfid/46570320385, CC BY 2.0. More than a thousand people died during this, one of the worst storms on record to have hit Africa. While no single flooding event can be attributed to climate change, it is undeniable that warmer oceans create conditions for hurricanes and cyclones to be stronger, bigger, and more frequent.
To combat climate change, politicians of several countries have started to enact laws to reduce greenhouse gas emissions and habitat destruction (Section 12.2.1). Many industries are also hard at work developing “greener” technologies to enable us to live more sustainable lives. Conservation biologists also play a crucial role in mitigating the negative impacts of climate change. In addition to highlighting the plight of the natural world to society at large, we could work towards reducing the loss of ecosystem services and preventing species extinctions. To accomplish this task, we need to identify which species and ecosystems are most sensitive to climate change and develop strategies that will ensure the continued persistence of as many sensitive species and their habitats as possible. The rest of this chapter is dedicated to methods we can employ to understand which species are sensitive, and how they may respond to climate change, while Chapters 10–15 discuss methods we can employ to better address climate change.
6.3.2 Climate change’s impact on terrestrial ecosystems
Aside from regional variations in temperature and precipitation, Earth’s surface will be a few degrees warmer in future than the temperatures we experience today. In effect, that means that today’s climatic zones will generally shift upslope in mountainous areas and towards the poles on lowlands, plains, and plateaus. To survive, climate-sensitive plants and animals will need to track these shifts so that they remain within their suitable climatic envelopes of temperature and precipitation.
Climate change on mountains
Species that live on mountains are at particular risk from climate change. Because temperatures decrease by roughly 0.65°C for every 100 m in elevation rise (known as temperature lapse rates), a 1°C increase suggests that climate-sensitive species living on a mountain would be displaced by at least 150 m (1.5 m/year) upslope between the years 2000 and 2100. Species that live on the lower slopes of mountains and are mobile enough to make such an adjustment may have opportunities to move to higher ground. However, species that live on or near peaks may have nowhere else to go as the world heats up, resulting in what biologists call mountain-top extinctions. While a mountain-top extinction has yet to be recorded in Africa, we have ample evidence to suggest that the region’s wildlife is vulnerable to it. For example, due to climate change, populations of some bird species endemic to the Cape Floristic region’s mountains have shrunk by 30% over the past two decades (Milne et al., 2015). Species inhabiting Tanzania’s Eastern Arc Mountains (Dimitrov et al., 2012), Albertine Rift (Ponce-Reyes et al., 2017), and the Guinean Forests of West Africa (Carr et al., 2014) appear to have experienced similar declines. Given these observations, it is only a matter of time before one of Africa’s mountain specialists follows the example of Costa Rica’s once abundant Monteverde golden toad (Bufo periglenes, EX), the first known amphibian extinction attributed to climate change (Crump et al., 1992).
Species that live on mountain peaks are vulnerable to climate change because they may have nowhere else to go as the world heats up.
Climate change in the lowlands
The response of species living in lowlands and on plains tend be more variable and complex than those living on mountains. While some species may only need to make minor range adjustments, researchers estimate that some African taxa may need to move 500 km (Barbet-Massin et al., 2009)—maybe even 1,000 km (Hsiang and Sobel, 2016)—to keep up with climate shifts. For species, such as Tanzania’s savannah birds that have already shifted their distributions by 200–300 km (Beale et al., 2013), adapting seems relatively easy thanks to their mobility and largely intact ecosystems. Unfortunately, the rate of climate change will likely outpace the ability for most species to adapt (Jezkova and Wiens, 2016; Wiens, 2016). For example, nearly 62% of Sub-Saharan Africa’s species are predicted to undergo range contractions (Hole et al., 2009), and 37% species are facing extinction if climate forecasts hold true (Thomas et al., 2004). Species living in Southern Africa’s Miombo Woodlands are even more vulnerable, where as many as 90% of amphibians, 86% of birds, and 80% of mammals face extirpation (Warren et al., 2018).
Species of tropical lowland forests and deserts are also highly vulnerable to shifting climates. Many tropical species have narrow tolerances for temperature and rainfall variation, while desert specialists may be at the limits of their physiological heat and desiccation tolerances (Figure 6.4). Consequently, even small changes in the climate of these two ecosystems may have major effects on reproduction, species distributions, and hence ecosystem composition (Box 6.2). One species already impacted is the nocturnal aardvark (Orycteropus afer, LC): a study in Southern Africa’s Kalahari Desert found over 80% mortality rates in this species during recent summers (Rey et al., 2017). The high levels of mortality in this species was attributed to above average temperatures, which subjected the animals to heat stress, leading to behavioural disruptions, declining body conditions, and eventually starvation. The impact of climate change on the aardvark is of concern because it is an ecosystem engineer: their burrows provide denning and refuge sites for multiple other species (Whittington-Jones et al., 2011).
Box 6.2 Desert Birds and Climate Change
1FitzPatrick Institute of African Ornithology, DST-NRF Centre of Excellence,
University of Cape Town, South Africa.
2DST-NRF Centre of Excellence at the FitzPatrick Institute,
Department of Zoology and Entomology,
University of Pretoria, South Africa.
3South African Research Chair in Conservation Physiology, National Zoological Garden,
South African National Biodiversity Institute,
Pretoria, South Africa.
susie.j.c@gmail.com
Deserts, with their extreme temperatures and scarce and unpredictable rainfall, are among the most inhospitable environments on the planet. To survive and breed in arid regions, organisms must minimise their energy and water requirements, and avoid exposure to potentially lethal temperatures. Birds are generally small and diurnal; and are therefore among the groups of animals most vulnerable to even small increases in air temperatures associated with climate change. Studies of the effects of temperature on arid-zone birds can thus be highly informative in terms of identifying new conservation challenges posed by global warming, developing mitigation measures, and understanding the management interventions that may become necessary during the 21st Century.
Daytime temperatures in many deserts regularly exceed avian body temperature, creating conditions under which birds can avoid lethal heat stroke only by dissipating heat via evaporation. But rapid rates of evaporation increase the risk of birds becoming lethally dehydrated. Desert birds thus face life-or-death decisions between avoiding hyperthermia by evaporative cooling versus avoiding lethal dehydration by minimising water losses. Mass mortality events occasionally take place during extreme heat waves when air temperatures exceed birds’ physiological tolerance limits. In Australia, for example, there are both historic and contemporary accounts of die-offs sometimes involving millions of birds. As Earth heats up under climate change, the risk of such die-offs in desert birds is expected to increase dramatically for the deserts of Australia and North America during the 21st Century (McKechnie and Wolf, 2010; Albright et al., 2017).
Africa’s arid regions are also experiencing significant temperature increases which are predicted to continue over the next several decades (Conradie et al., 2019). Under these conditions, the impact of air temperature on avian physiology can be mediated by behaviour. Birds employ a trio of behavioural adjustments to manage heat load and keep their body temperatures within safe limits. These include shade-seeking, reducing activity to minimise metabolic heat production, and gaping the beak (panting, sometimes accompanied by gular flutter) to facilitate respiratory evaporative cooling (Figure 6.B). Although these behaviours can buffer birds against physiological costs of high temperature, they carry subtle but important costs of the own, notably via their impact on birds’ ability to forage.
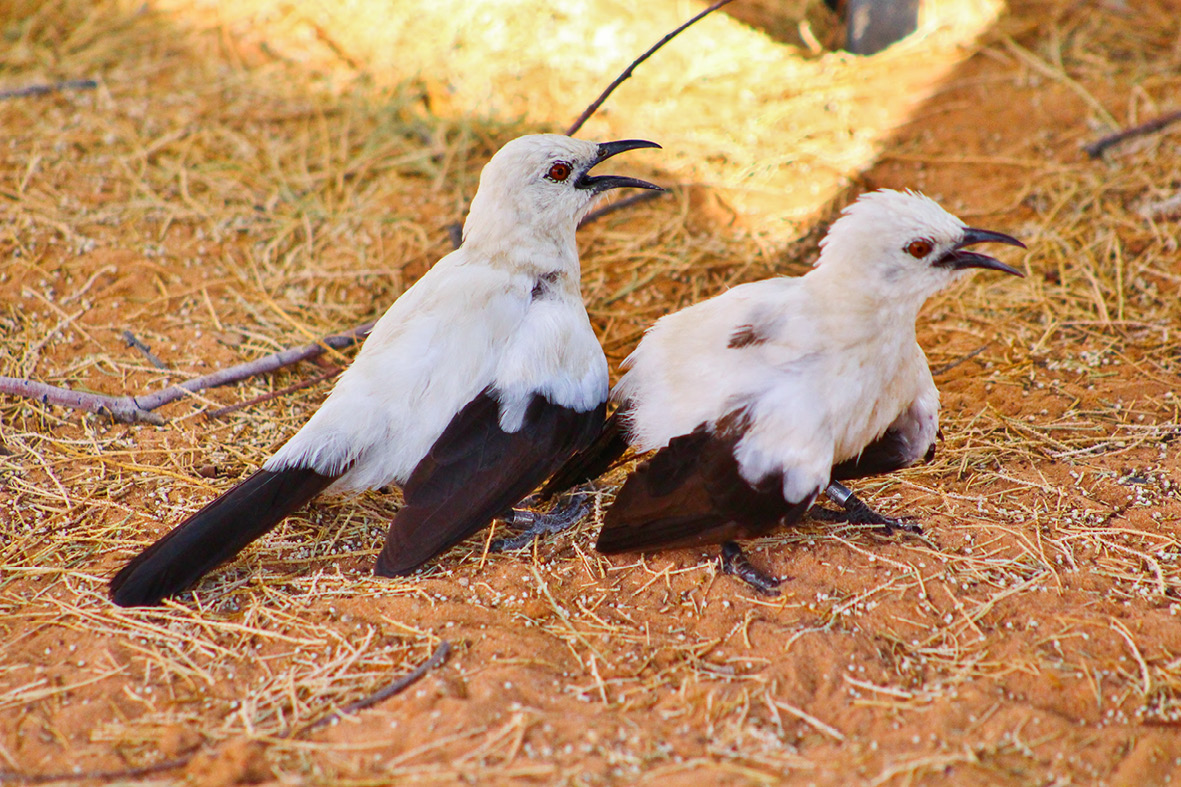
Figure 6.B A pair of southern pied babblers (Turdoides bicolor, LC), an endemic of Southern Africa’s arid savannahs, gaping their beaks to facilitate respiratory evaporative cooling during a particularly hot summer afternoon. Photograph by Nicholas Pattinson, CC BY 4.0.
For desert birds, foraging is critically important for maintaining both energy and water balance, as most species obtain all their water from food. Reduced activity almost inevitably means reduced food intake via impacts on time available for foraging. Seeking shade also carries costs: for some species, returns on foraging effort in shaded locations are significantly lower than in the sun (e.g. Cunningham et al., 2013). Finally, respiratory evaporative cooling can severely restrict the ability of actively-foraging birds to acquire food due to mechanical constraints on simultaneously gaping the bill and using it for prey capture and handling (e.g. du Plessis et al., 2012).
Under climate change, the implications of these behavioural trade-offs between foraging and thermoregulation are non-trivial. Inability to balance water and energy budgets mean birds progressively lose body condition during heat waves (du Plessis et al., 2012). Compromised foraging also affects birds’ capacity to provision offspring, resulting in reduced nest success and/or smaller, lighter fledglings which may struggle to survive and recruit into the breeding population (e.g. Cunningham et al., 2013, Wiley and Ridley, 2016).
Successfully balancing the trade-offs between foraging and thermoregulation, and between hyperthermia and dehydration, is the secret to success for birds in hot places. As the climate warms, achieving this balance will become ever more challenging. Sublethal behavioural costs of keeping cool kick in at temperatures cooler than those promoting mass mortalities. In some parts of the world, such as Southern Africa, the loss of birds from desert ecosystems may therefore occur through the insidious whittling away of fitness and weakening of populations (Conradie et al., 2019) before we even witness the dramatic die-off events for which Australia is already infamous.
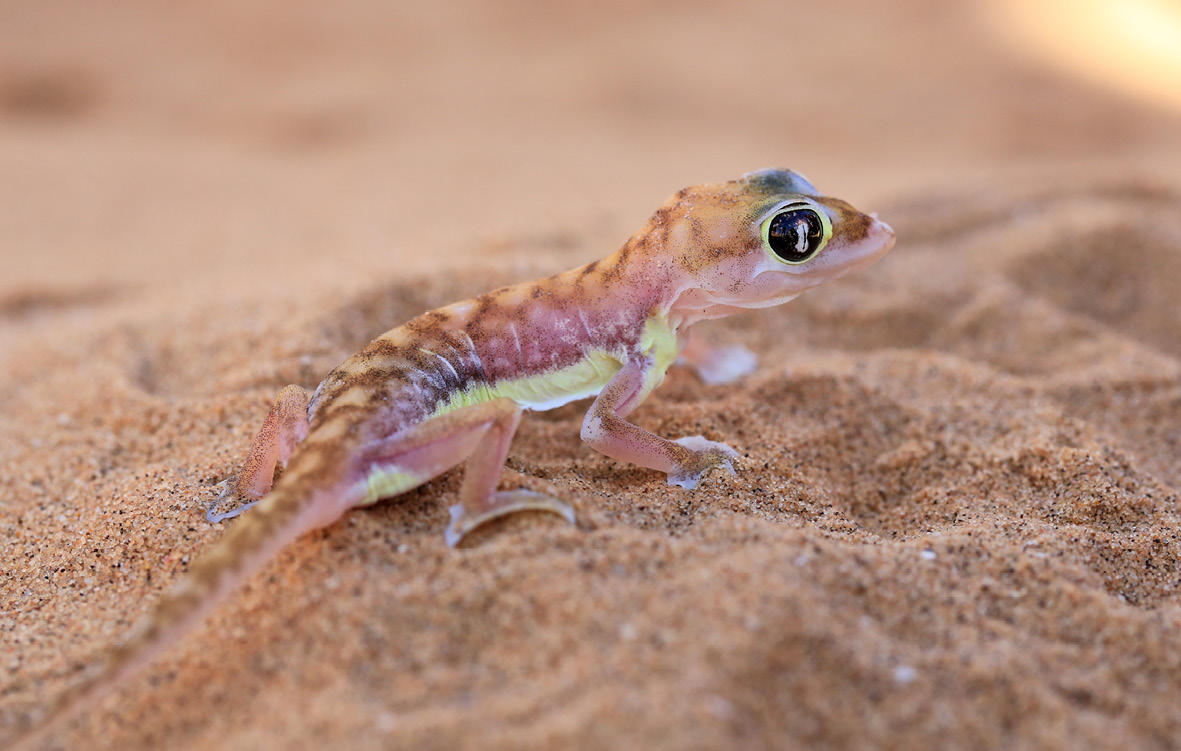
Figure 6.4 The Namib sand gecko (Pachydactylus rangei), endemic to Namibia’s Namib Desert, survives the scorching heat by being nocturnal and burrowing into loose sand with its webbed toes. Warmer conditions under climate change may make it much harder for the gecko and other desert species, operating at the limits of their physiological tolerances, to survive. Photograph by Marije Louwsma, https://www.inaturalist.org/observations/18594993, CC BY 4.0.
An additional concern for lowland ecosystems is that climate change will likely lead to the creation of novel (i.e. hotter) ecosystems unlike any others currently on Earth (Williams et al., 2007). These changes will lead to biotic attrition. The gradual impoverishment of biological communities of lowland ecosystems as species either go extinct or move away while tracking their climatic envelopes. What is not clear is how the niches left open by the net loss of species, and newly created niches in the novel ecosystems, will be filled. The most likely scenario is that more tolerant, generalist species will fill the empty niches. However, with the inevitable loss of some species, combined with the decoupling of important biological interactions (discussed below), some functions and services associated with lowland ecosystems are likely to eventually collapse. It is important to note that tropical lowland forests and deserts are by no means the only ecosystems vulnerable to biotic attrition. For example, researchers have found that even mild warming would expose the Ethiopian Highlands to biotic attrition (Kreyling et al., 2010).
Climate change and dispersal limitations
Across many diverse ecosystems, a great number of species are threatened by climate change because of their poor dispersal abilities. Because they lack appropriate dispersal mechanisms, species, such as slow maturing plants (Foden et al., 2007), mosses, and flightless insects may simply not be able to keep up with changing climatic conditions. The impacts of climate change on Africa’s dispersal-limited species can already be seen. For example, the once abundant Aldabra banded snail (Rhachistia aldabrae, CR) is today so rare that this Lazarus species (Figure 6.5) was once believed to be extinct due to climate change (Battarbee, 2014). There are also fears that successive droughts in the Cape Floristic Region may have recently driven a rare sorrel species (Oxalis hygrophila, CR) to extinction (Zietsman et al., 2008). Next might be the cave katydid (Cedarbergeniana imperfecta, CR) and Marais’ lace-winged katydid (Pseudosaga maraisi, CR); these highly threatened insects count among Africa’s very few cave specialists, and yet, by living in highly restricted and restrictive ecosystems, they face major challenges in adapting to climate change (Bazelet and Naskrecki, 2014). Dispersal limitations will also greatly affect terrestrial species living on oceanic islands, which will find it near impossible to track their climatic niches as it moves over the ocean. One such species is Cabo Verde’s Raso lark (Alauda razae, CR); with a population size that fluctuates in response to rainfall, climate change induced drought conditions have pushed this bird to the brink of extinction in recent years (BirdLife International, 2016).
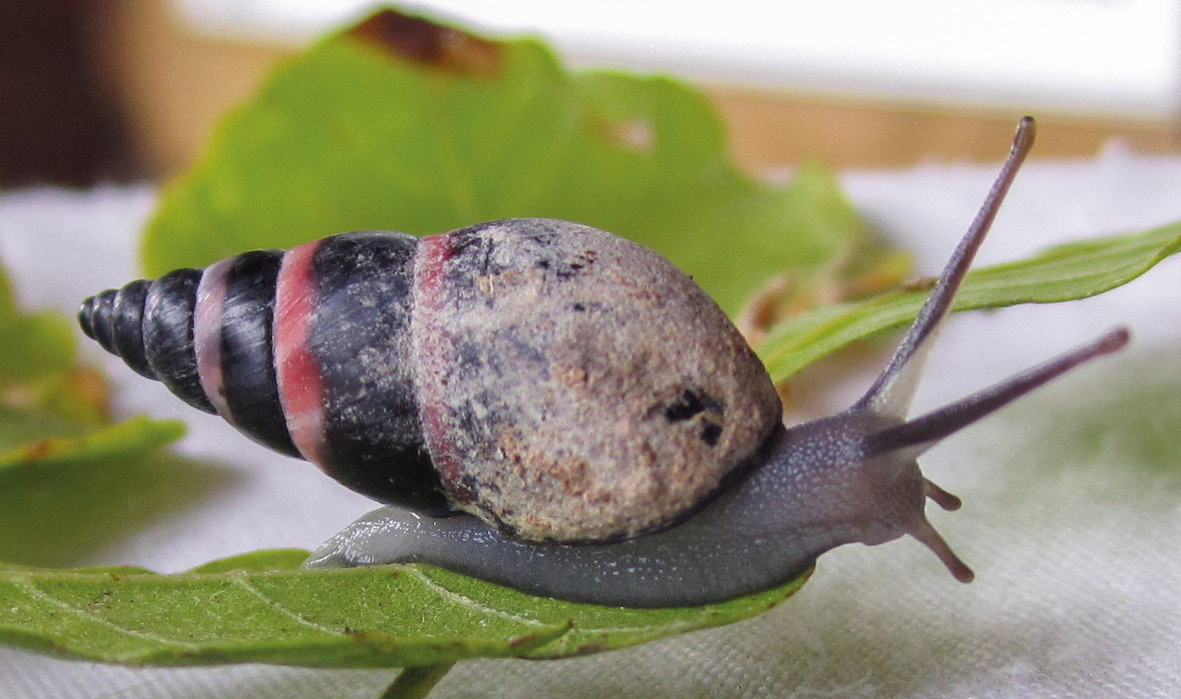
Figure 6.5 The Seychelles’ Aldabra banded snail was once thought to be one of the world’s first species pushed to extinction by climate change. Luckily, a small isolated population has been discovered, offering conservation biologists a second chance to ensure this species’ survival. Photograph by Catherina Onezia/Seychelles Islands Foundation, CC BY 4.0.
Climate change and biological interactions
Species that are highly mobile are not entirely spared from the negative impacts of climate change. Consider migratory species for a moment. The same way the musicians of an orchestra rely on a conductor to remain synchronised, migratory species rely on environmental cues, such as daylength and temperature, to decide when they need to start moving from one area to the next. But because different species rely of different environmental cues to time their life cycles (e.g. breeding), not all species will adjust to climate change at the same rate. There is consequently a high likelihood that climate change will disrupt these synchronous movements that the animal kingdom has developed over thousands of years (Renner and Zohner, 2018). This disruption of timed aspects of species’ life cycle, such as migration and breeding, is called phenological mismatch or trophic asynchrony. Researchers have already seen signs of phenological mismatch: some migratory birds that overwinter in Africa have started to migrate to their European breeding grounds at earlier dates than before (Both et al., 2006; Vickery et al., 2014). If these trends hold, they may soon start breeding before peak food availability, which could lead to lower fitness of offspring.
We can already see evidence of how climate change is disrupting migrations and mutualistic relationships that were developed over thousands of years.
Resident species are also vulnerable to phenological mismatch. While these species might not be known for large-scale movements around the globe, they may still have to adjust their ranges to keep track of their climatic niches. Considering the improbability of different species will adapt at the same pace, there is thus a danger that important mutualistic relationships might be pulled apart these during range adaptations. This is of concern for species with specialised feeding niches, as seen in some pollinators. For example, studies from South Africa have shown how necessary range adjustments under climate change threaten both sunbirds—which show low adaptability (Simmons et al., 2004)—and their host plants, if specialised pollinator niches are left vacant (Huntley and Barnard, 2012). Extinctions arising from this decoupling of mutualistic relationships are referred to as coextinction (Koh et al., 2004), while a series of linked coextinctions is called an extinction cascade (Section 4.2.1).
Climate change and reptiles
One may think that reptiles—often seen basking on sun-drenched rocks to obtain active body temperatures—may benefit from climate change. Yet, as a group, they are also expected to suffer under climate change. One reason is because many reptiles will also have to adapt their ranges to shifting climates (Houniet et al., 2009). Even more important, climate change will increase reptiles’ vulnerability to demographic stochasticity (Section 8.7.2). Many reptiles—and some fish—have their sex determined by temperature during embryonic development, with warmer temperatures often leading to more females (Valenzuala and Lance, 2004). In general, females regulate their offspring’s sex ratios by fine-scale breeding site selection. Under climate change, however, it might be harder for the females to find breeding sites with suitable microclimates. This situation is of concern at South Africa’s iSimangaliso Wetland Park, where Nile crocodiles (Crocodylus niloticus, LC) are already struggling to find suitable breeding sites due to microclimate changes caused by invasive plant encroachment (Leslie and Spotila, 2001). Those species unable to adopt new mechanisms to control for offspring sex ratio bias may eventually go extinct, even under relatively small temperature shifts (Sinervo et al., 2010).
6.3.3 Climate change’s impact on freshwater ecosystems
With Africa’s freshwater ecosystems already strained by the demands of a growing human population, freshwater biodiversity will face several additional stressors associated with climate change. Climate change will impact water temperature, flow volume, and flow variability. Because these variables are three primary predictors of freshwater ecosystem composition (van Vliet et al., 2013; Knouft and Ficklin, 2017), it is expected that climate change will greatly affect freshwater ecosystem composition and functioning in the coming decades.
Warmer rivers and streams
Climatologists and hydrologists predict that freshwater ecosystems will generally experience temperature increases under climate change. These changes are already evident in Africa: for example, Lake Albert on the DRC-Uganda border, and Zambia’s Lake Mweru Wantipa, have experienced surface temperature increases of 0.62°C and 0.56°C respectively over the past decade (O’Reilly et al., 2015). Like their terrestrial counterparts, many freshwater species are sensitive to temperature shifts (e.g. Reizenberg et al., 2019). Warmer water also holds less dissolved oxygen, and increased pollutant toxicity (Whitehead et al., 2009). In addition, longer growing seasons and higher water temperatures will lead to a general increase in primary productivity and decomposition rates, which in turn will lead to increased nutrient loads, algae blooms, and eutrophication (Whitehead et al., 2009). All these factors will force many freshwater species—even those not sensitive to temperature shifts—to adjust their ranges to keep track of suitable conditions. Many of these adjustments will be impeded by habitat fragmentation, notably by dams and other human constructs that block suitable dispersal pathways. As an additional complication, many aquatic organisms cannot travel overland, so are naturally limited to adjust their ranges along the rivers and streams in which they live. But the orientation of these rivers and streams may not follow suitable thermal isolines: consider a cold-water species that needs to disperse to higher elevation—and hence upstream—as its climate niche moves higher up a mountain. For some freshwater species, the impediments to adjusting their ranges as necessary may be insurmountable.
Climate change will alter water temperature, flow volume, and flow variability, the three primary predictors of freshwater ecosystem composition.
Changing flow regimes
Changing precipitation levels will have several impacts on freshwater ecosystems, particularly as it relates to changes in their flow regimes (Thieme et al., 2010; Knouft and Ficklin, 2017). For example, areas that are undergoing decreased precipitation will experience decreased runoff and increased drying of wetlands and small streams, while areas with increased precipitation will experience increased storm surges and flushing. These changes, together with the impacts of increased water extraction rates and evapotranspiration under a warmer world, will cause significant changes in water levels, flow rates, sediment loads, water turbidity, and the structure of the physical environment. With an estimated 80% of Africa’s freshwater fishes predicted to experience significant flow regime changes (Thieme et al., 2010), the region will likely see substantial changes in the composition of freshwater communities in the coming decades.
Given these multiple stressors, there is a reasonable expectation that many freshwater species will go extinct or face significant population declines and range shifts over the next decades. These changes are of major concern in Africa, where so many people depend on fish and related natural resources for their livelihoods. Communities in Uganda, Malawi, Guinea, and Senegal are already finding it more difficult to meet their nutritional needs due to climate-induced freshwater fish declines (Allison et al., 2009). Also, at Lake Tanganyika—which supplies 20–40% of the surrounding countries’ dietary protein—fish yields have decreased by 30% in recent years, also attributed to climate change (O’Reilly et al., 2004).
6.3.4 Climate change’s impact on marine ecosystems
Like tropical forests, the world’s oceans have historically provided a relatively stable environment in which marine organisms have evolved. While this stability promotes species diversity, it also leaves marine species more vulnerable to environmental changes. In fact, a recent study found that cold-blooded marine species are twice as vulnerable to the impacts of warmer oceans than their terrestrial counterparts (Pinsky et al., 2019). In addition to the impacts of storm surges (Figure 6.6) and ocean warming (which leads to rising sea levels and ocean deoxygenation), marine organisms also must deal with ocean acidification. These threats will likely have impacts like those faced in terrestrial and freshwater ecosystems, including range adjustments, biotic attrition, and decoupling or important interactions. Below we discuss the mechanisms that will lead to some of these changes in more detail.
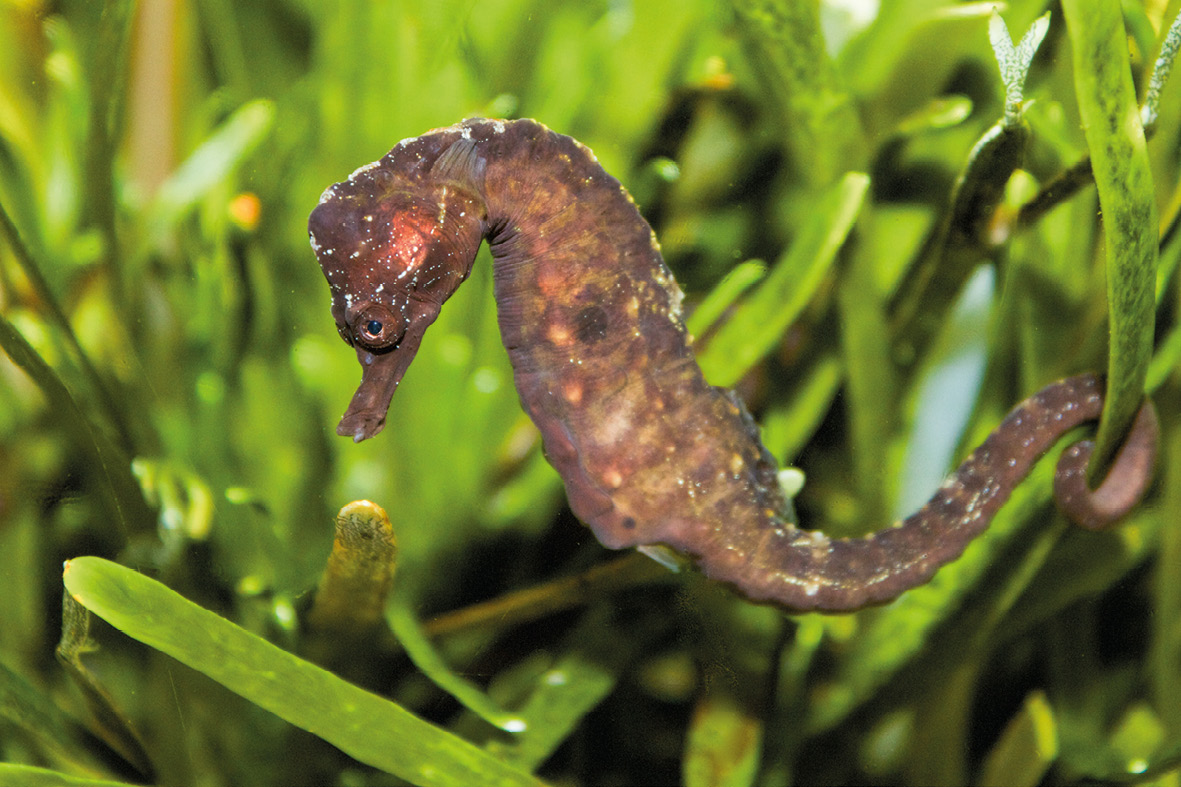
Figure 6.6 Many species living in low-lying coastal regions may be pushed to extinction by more frequent cyclones/hurricanes, storm surges, and sea level rise resulting from climate change. One such example is the Knysna seahorse (Hippocampus capensis, EN); biologists have attributed the deaths of thousands of these unique animals to temperature fluctuations and flooding events that altered parts of their highly restricted range in South Africa (Pollom, 2017). Photograph by Brian Gratwicke, https://www.flickr.com/photos/briangratwicke/7108174613, CC BY 2.0.
Ocean acidification
As discussed earlier, human activities release massive amounts of CO2 into the atmosphere each day. Although forests and other plant communities get considerable attention for CO2 sequestration, the world’s oceans also play a key role in keeping Earth’s carbon balance in check. In fact, the world’s oceans absorb an estimated 20–25% of our current CO2 emissions (Khatiwala et al., 2009). Now, with more atmospheric CO2 available, oceans absorb more carbon, which dissolves in seawater as carbonic acid. While this absorption may slow climate change, it also increases the acidity (i.e. lowing the pH levels) of the world’s oceans. This process—known as ocean acidification—has several consequences that may directly and indirectly kill marine organisms. For example, it inhibits the ability of coral animals to deposit the calcium used to build their reefs’ structure (Mollica et al., 2018), and prevents shellfish from accumulating adequate amounts of calcium carbonate to develop shells strong enough for survival (Branch et al., 2013). Ocean acidification also disturbs predator-prey dynamics by impairing the senses of prey species (Leduc et al., 2013), and compromising the ability of marine creatures to communicate with conspecifics (Roggatz et al., 2016).
Climate change is causing sea level rise and increased seawater temperatures, with broad implications for marine ecology and people living in coastal areas.
Sea level rise
Over the past 30–40 years, ocean surface temperatures have warmed by about 0.64°C (NOAA, 2016).Ocean warming has several implications, the most well-known being sea level rise, caused by the thermal expansion of ocean water combined with the released water from melting glaciers and polar ice caps. Current predictions suggest that sea levels in Sub-Saharan Africa will rise by 0.2–1.15 m over the next 100 years, compared to 2005 levels (Serdeczny et al., 2017). As the oceans creep further inland, the extent of low-lying coastal ecosystems such as rocky shores or sandy beaches will shrink, and so also the sizes of the wildlife populations living in those areas. The extinction of Australia’s Bramble Cay melomys (Melomys rubicola, EX)—the world’s first documented mammalian extinction caused by anthropogenic climate-change—has been attributed to sea level rise (Gynther et al., 2016).
Coral bleaching
The incredible diversity of corals reef ecosystems is attributable to the relative stability of tropical oceans. Because of this stability, individual coral species have adapted to very specialized niches. Many corals thus tolerate only narrow ranges in temperature, sunlight levels, water opacity, and nutrient loads. Climate change is disrupting this stability, by changing the temperature (ocean warming), depth (sea level rise), sediment and nutrient loads (increased erosion and runoff) of the environments where corals live. These changes are leading to a breakdown of critical mutualistic relationships between photosynthetic algae and corals. In the process, corals also lose their vibrant colours, revealing the corals’ ghostly white skeletons, hence the name coral bleaching (Figure 6.7). This relationship breakdown deprives the corals of essential carbohydrates they obtain from the algae, causing the corals to starve to death if the stressful conditions continue for a prolonged time.
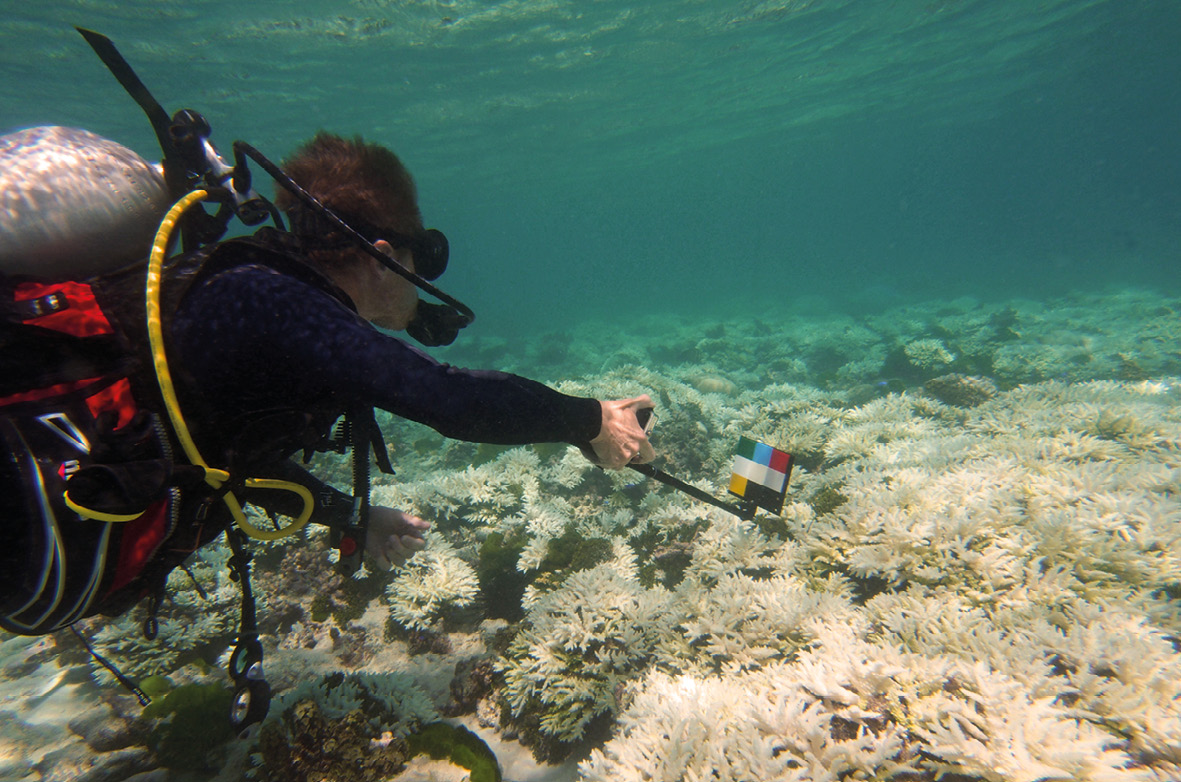
Figure 6.7 A marine biologist surveys bleached corals in Curieuse Marine National Park, Seychelles. Bleaching events occur when heat stress kills corals, leaving only white skeletons where a once-vibrant coral reef community existed. Photograph by Emma Camp, CC BY 4.0.
Africa’s tropical oceans have experienced extensive coral bleaching events in recent years. For example, parts of Tanzania and Kenya have seen over 80% of their corals affected (McClanahan et al., 2007; Chauka, 2016). Coral bleaching also affects other species associated with coral reefs. For example, in the Seychelles, where coral bleaching at 70–99% of reefs was observed, butterfly fish exhibited a breakdown in territorial behaviour, making it hard for them to breed and feed (Samways, 2005). In Zanzibar, Tanzania, eroded fish communities showed little signs of recovery multiple years after a bleaching event (Garpe et al., 2006).
Ocean deoxygenation
Marine fish and invertebrates rely on dissolved oxygen that enters the water either through the atmosphere, or by photosynthetic plankton. But because warmer water absorbs less oxygen, scientists predict that some areas of the ocean will see a 3–6% drop in dissolved oxygen concentrations under climate change (IPCC, 2014). This process, known as ocean suffocation or ocean deoxygenation (Ito et al., 2017), will leave parts of the ocean unsuitable for marine fishes and invertebrates. The impact of ocean deoxygenation will also be felt by economically important fisheries, notably along West Africa (Long et al., 2016) where climate change is predicted to lead to fisheries-related economic losses upwards of US $311 million each year (Lam et al., 2012).
6.3.5 Climate change interacts with habitat loss
Habitat loss and climate change each cause negative impacts on biodiversity; however, these threats also interact to have an overall larger negative impact than the sum of these threats independently. Prominently, because of habitat loss, many species will be unable to adequately adjust their ranges to keep track of their shifting climatic niches. For example, some species might not be able to adapt their ranges because suitable habitat in their future ranges will be destroyed by human activity.
Climate change interacts with habitat loss, by impeding species’ ability to adapt, and by bringing dispersing wildlife into conflict with humans.
Range-shift gaps describes a habitat gap that prevents a species from dispersing from its current to future ranges (Figure 6.8). These gaps, which may occur naturally or because of habitat fragmentation, may also impede range adjustments under climate change. While the impact of range-shift gaps is an active area of research, it is expected that mountain-top species may be inherently vulnerable to range-shift gaps, particularly if they are unable to first disperse downslope before they can reach climatically suitable locations at higher elevation elsewhere. For example, over 60% of herbaceous plants living on Ethiopia’s Arsi Mountains might face range-shift gaps soon (Mekasha et al., 2013). But even highly mobile species might be vulnerable, with many African birds expected to face range-shift gaps as they adjust their ranges (La Sorte et al., 2014).
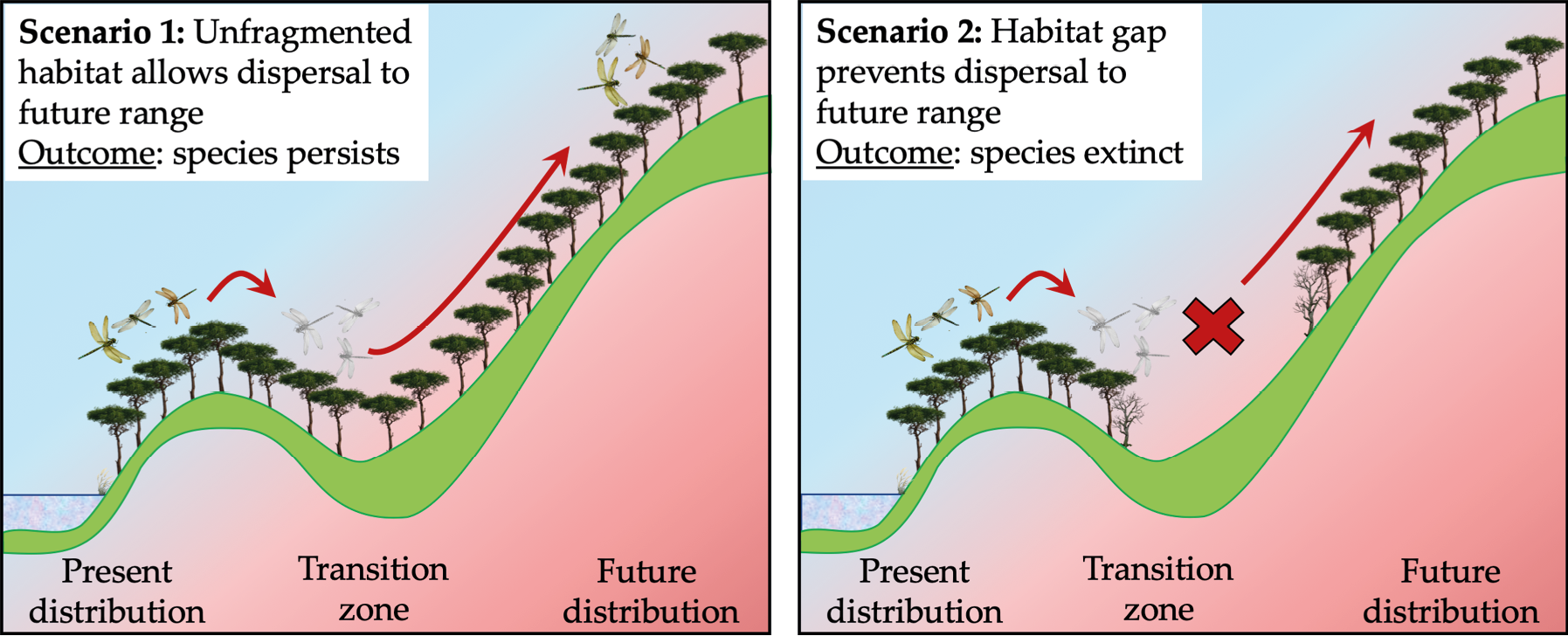
Figure 6.8 Hypothetical example of a species adjusting its range to climate change along unfragmented habitat (Scenario 1) and a species unable to adjust its range due to a range-shift gap (Scenario 2). In Scenario 1, the species persists; in Scenario 2 the species goes extinct because a gap in available habitat prevents dispersal into suitable areas. CC BY 4.0.
Habitat loss and climate change are also expected to exacerbate human-wildlife conflicts (Section 14.4). Sub-Saharan Africa will face losses of up to 2.5 million km2 in arable land between 2010 and 2100 (Zabel et al., 2014). These losses will see even more natural ecosystems converted for agriculture which, in turn, will further increase competition among and between humans and wildlife for resources such as food, water, and suitable habitat (Serdeczny et al., 2017). As the human footprint expands across Earth, agriculture and infrastructure will impede the ability of specialist species to find food and adapt to changing conditions, while generalist species will be forced into agricultural lands and nearby human habitation as they search for resources and/or disperse across the landscape. Such a scenario will likely exacerbate human-wildlife conflict in areas like Kenya’s Amboseli region, where lions living in fragmented ecosystems with diminishing natural prey populations are increasingly prone to wandering beyond protected area boundaries into ranching areas in search of food (Tuqa et al., 2014).
6.4 Beneficiaries of Climate Change
To be clear, not all species will suffer equally from climate change. In fact, there are some species that will be resilient, and others that will even benefit from a warming world. Primary among the beneficiaries are plants in the northern areas of Europe, Asia, and North America (Zabel et al., 2014), and to a lesser extent in southern South America and New Zealand. In these areas, plants will benefit from longer growing seasons (earlier springs and shorter winters) and increased CO2 concentrations (which will increase photosynthesis rates).
Generalist species with high genetic diversity and that reproduce quickly are likely to benefit from climate change. Many species that exhibit these traits carry diseases and are agricultural pests.
Closer to home, a variety of African species are also expected to benefit from climate change. These include generalist species currently limited by interactions with localised specialists that are—at least at present—better competitors for limiting resources. Some tropical species may thrive as their habitats become hotter and wetter. Species with high genetic diversity that reproduce quickly (allowing for rapid adaption to environmental changes) are also likely to benefit. Unfortunately, many species that exhibit these traits carry diseases (Box 6.3) and are agricultural pests (Serdeczny et al., 2017). For example, populations of the coffee berry borer (Hypothenemus hampei)—Africa’s most notorious coffee pest—are expected to greatly increase in a warmer world (Jaramillo et al., 2011). This growing threat is particularly worrying given that higher temperatures have already reduced coffee harvests in countries such as Tanzania by as much as 50% (Craparo et al., 2015).
Box 6.3 Habitat Alteration, Climate Change, and Mosquito-Borne Diseases
Center for Tropical Research,
UCLA Institute of the Environment and Sustainability,
Los Angeles, CA, USA.
kynjabo@hotmail.com
With unprecedented climate change looming, mosquito-borne diseases, including malaria and dengue fever, will impact humans and wildlife in novel and unpredictable ways. While climate change is global in nature, changes due to habitat alteration are occurring more rapidly on a local scale, and are having significant effects on mosquito-borne diseases (Figure 6.C). For example, destruction of Peruvian rainforests unleashed more than 120,000 cases of malaria in the late 1990s, compared to fewer than 150 nine years earlier (Vitor et al., 2006).
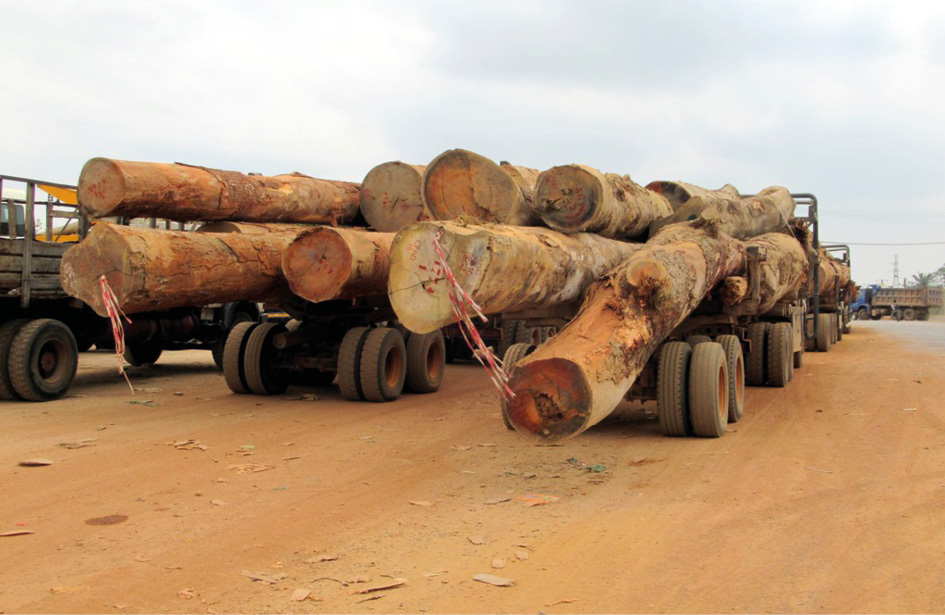
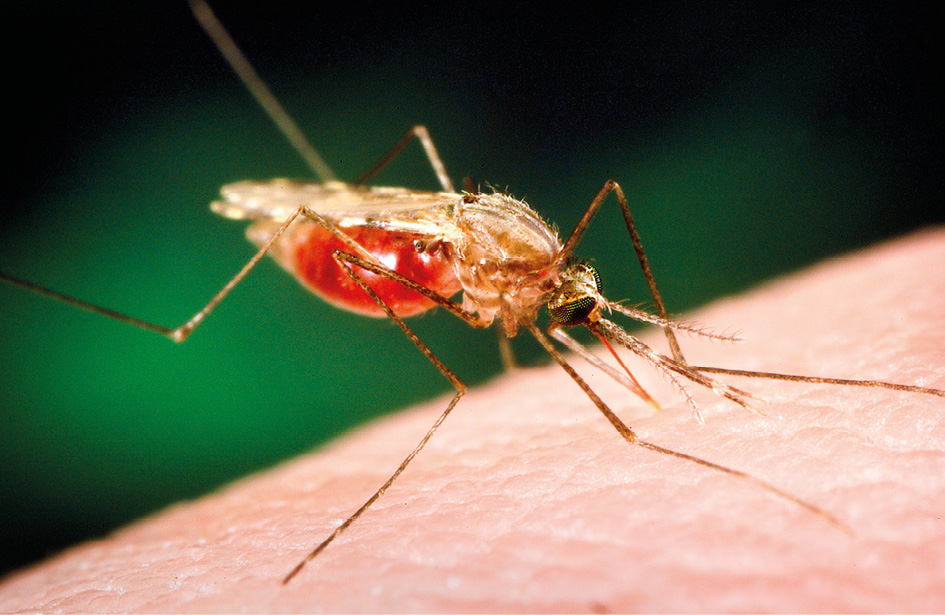
Figure 6.C (Top) Trucks transporting recently logged trees in Gabon. Photograph by David Stanley, https://www.flickr.com/photos/davidstanleytravel/46170117302, CC BY 2.0. (Bottom) Anopheles funestus, one of the most important vectors of malaria in Africa. Photograph by USCDCP, CC0.
The rainforests of the Congo Basin harbour roughly 20% of all known plant and animal species on Earth. Yet, habitat alteration continues at an alarming rate (Harris et al., 2012). Exacerbating these threats is the fact that Africa (Boko et al., 2007), and Central Africa in particular (McClean et al., 2006), are predicted to be some of the most severely affected by climate change. Predicted temperature increases would lead to longer seasons of malaria transmission and a 5–7% extension of the disease into higher latitudes (Craig et al., 1999, Boko et al., 2007). Coupled with projected population growth, climate change would nearly double the number of people at risk of infection from dengue fever by 2080. This is concerning because Africa is particularly vulnerable to environmental changes due to its limited adaptive capacity, widespread poverty, and low levels of development.
How then, will habitat alteration and climate change affect mosquito-borne diseases such as malaria? The relationship between disease transmission, habitat alteration, and climate change is complex. Though deforestation increases the risk of disease transmission (Vitor et al., 2006), different malaria-carrying mosquitoes (Anopheles spp.) are adapted to different microclimates. Ironically, our multi-faceted ecosystems both play the role of maintaining transmission cycles with cross-infections to humans and regulating those cycles while controlling spill-over into human populations. The balance between these factors is influenced by the availability of suitable habitat for mosquitoes and of reservoir hosts of infection. In an ideal world, transmission cycles are regulated by density-dependent processes such as acquired immunity to infectious diseases, and by limits on the carrying capacity of the environment to support insects and hosts.
Altered natural habitats and possible increases in disease transmission from animals to people also increase potential risks of new pathogens adapting to human hosts. Only about 2,000 of an estimated 1 million unique viruses carried by wild vertebrate species with potential zoonotic threats have been described. For example, when a lentivirus of chimpanzees first jumped into humans in the 1930s, not many people died. But the disease carved a foothold in the rapidly growing African city of Kinshasa in DRC and evolved into a form that efficiently preyed upon humans. More than 78 million people were infected between 1981 and 2015. To date, the disease it causes, AIDS, has killed more than 39 million people, while another estimated 37 million people are living with HIV.
Today, habitat alteration, such as deforestation, is not only driving species extinct and emitting lots of climate-changing carbon dioxide, it is also increasing opportunities for mosquito-borne diseases, such as malaria and dengue, to infect more humans in new places. Technological advances, including mathematical and computer modelling, genomics, and satellite tracking, will hopefully allow us to predict future disease outbreaks better. But we can also reduce outbreak opportunities by taking better care of our environment.
One group of species currently threatened with extinction that may benefit from a warmer world is marine turtles. Researchers working on Cabo Verde speculate that the island nation’s loggerhead turtle (Caretta caretta VU) populations will benefit from an increasing female-biased sex ratio (as expected under warmer conditions) given that a single male can breed with several females (Laloë et al., 2014). However, the researchers note that this population requires continued monitoring as insurance against demographic stochasticity (Section 8.7.2) that may become a larger threat under climate change.
Climate change has the potential to greatly restructure the world’s ecosystems, ecosystem services, and national economies.
6.5 The Overall Impact of Climate Change
It should be clear to anyone that climate change has the potential to greatly restructure the world’s ecosystems, ecosystem services, and national economies. Many coastal areas will experience rising sea levels and increased flooding, while inland areas may experience desertification and less favourable crop growing conditions. Poor Africans will suffer the consequences disproportionately because of their limited mobility, high dependence on ecosystem services, and general lack of disaster management infrastructure (Serdeczny et al., 2017).
If we are to mitigate the far-reaching impacts of climate change, we must carefully monitor and study changes in biological communities and ecosystem functioning, and how they relate to changes in climate and other stressors. While we may lose some species in a warmer world, we can also prevent many extinctions with pro-active wildlife management (Section 11.4). It is likely that many existing protected areas will no longer preserve some of the rare and threatened species that currently live in them (Hole et al., 2009; Smith et al., 2016, but see Beale et al., 2013), necessitating careful planning when establishing new protected areas (Section 13.7.2). Even if climate change is not as severe as predicted, the steps we take now to safeguard biodiversity can only help in future.
In 2007, the world economy was close to collapse because of the misdeeds of the financial services industry. Considering climate change’s record in causing societal disruption and suffering, and our increasingly globalised world (in which regional disruptions are felt much wider than before), politicians are rightfully concerned about our ability to adapt to a widespread restructuring of the world’s natural resources (Dietz et al., 2016). While the consequences of climate change are closely associated with the environmental sciences, it is truly, at its core, a human rights concern.
The widespread and dramatic impacts of climate change rightfully deserve much attention. But it is also important to remember that we continue to destroy habitat at a massive scale and increasing pace, and this loss of habitat is currently the main cause of species extinctions. The highest priorities for conservation must continue to be the preservation of healthy, intact, and connected ecosystems, and the restoration of degraded ecosystems. These actions will simultaneously reduce the impacts of climate change, by reducing carbon emissions, increasing carbon sequestration, and giving wildlife more opportunities to adjust their ranges, in their own time, as the world’s climate changes.
6.6 Summary
- While climate change is often thought of as a future challenge, we can already see its impacts today, as shown by record-high temperatures and changing rainfall patterns. These changes are happening because human activities release large amounts of greenhouse gases into the atmosphere on a daily basis.
- Habitat loss contributes to climate change directly through the destruction of complex ecosystems (i.e. carbon sinks) which releases stored CO2, and indirectly through the loss of vegetation that would otherwise sequester CO2 from the atmosphere.
- Some climatic shifts are predicted to be so rapid in coming decades that many species will be unable to adjust their ranges to keep up with environmental changes. Species with dispersal limitations, special habitat requirements, and important mutualistic relationships are at especially high risk of extinction.
- Mitigating the negative impacts of climate change will require an international multi-pronged approach that includes ecosystem protection and restoration, direct species management, and legislative action.
- Species are seldom exposed to only one threat; rather, different threats interact with climate change so that their combined impact is greater than their individual effects. A successful conservation strategy needs to deal with these threats collectively.
6.7 Topics for Discussion
- Think of any particular ecosystem in your region. How do you think climate change will impact that ecosystem? What single measure do you think can be implemented to reduce the impact of climate change on that ecosystem? Can you think of the resources you will need to implement that measure?
- Which groups of people and wildlife in Africa do you think will benefit the most from climate change and why? Who do you think will suffer the most and why?
- How should we deal with species that have nowhere to go under climate change? Should we let them go extinct? What if it is an economically important species, like one that supports an important fishery or ecotourism industry? What do you think are our best options to save such species?
6.8 Suggested Readings
Allison, E.H., A.L. Perry, M.-C. Badjeck, et al. 2009. Vulnerability of national economies to the impacts of climate change on fisheries. Fish and Fisheries 10: 173–96. https://doi.org/10.1111/j.1467-2979.2008.00310.x African fisheries are highly vulnerable to climate change.
Dietz, S., A. Bowen, C. Dixon, et al. 2016. ‘Climate value at risk’ of global financial assets. Nature Climate Change 6: 676–79. https://doi.org/10.1038/nclimate2972 Climate change will cause financial losses of up to US $24 trillion.
Hole, D.G., S.G. Willis, D J. Pain, et al. 2009. Projected impacts of climate change on a continent-wide protected area network. Ecology Letters 12: 420–43. https://doi.org/10.1111/j.1461-0248.2009.01297.x In coming decades, many species will not be able to survive in their present locations because of climate change.
IPCC. 2014. Climate Change 2014: AR5 Synthesis Report (Geneva: IPCC). https://www.ipcc.ch/report/ar5/syr Comprehensive presentation of the evidence for global climate change, along with predictions for the coming decades.
Jaramillo J., E. Muchugu, F.E. Vega, et al. 2011. Some like it hot: The influence and implications of climate change on coffee berry borer (Hypothenemus hampei) and coffee production in East Africa. PLoS ONE 6: e24528. https://doi.org/10.1371/journal.pone.0024528 Some important crop pests will benefit from a warming world.
La Sorte, F.A., S.H.M. Butchart, W. Jetz, et al. 2014. Range-wide latitudinal and elevational temperature gradients for the world’s terrestrial birds: Implications under global climate change. PLoS ONE 9: e98361. https://doi.org/10.1371/journal.pone.0098361 African birds are particularly vulnerable to climate change.
Niang, I., O.C. Ruppel, M.A. Abdrabo, et al. 2014. Africa. In: Climate Change 2014: Impacts, Adaptation, and Vulnerability, ed. by V.R. Barros, et al. (Cambridge: Cambridge University Press). https://www.ipcc.ch/site/assets/uploads/2018/02/WGIIAR5-Chap22_FINAL.pdf A review of climate change impacts across Africa.
Thieme, M.L., B. Lehner, R. Abell, et al. 2010. Exposure of Africa’s freshwater biodiversity to a changing climate. Conservation Letters 3:324–31. https://doi.org/10.1111/j.1755-263X.2010.00120.x Climate change will have wide-ranging impacts on Africa’s freshwater ecosystems.
Wiens, J.J. 2016. Climate-related local extinctions are already widespread among plant and animal species. PLoS Biology 14: e2001104. https://doi.org/10.1371/journal.pbio.2001104 Hundreds of species have already been subjected to local extinctions due to climate change.
Bibliography
Albright, T.P., D. Mutiibwa, A.R. Gerson, et al. 2017. Mapping evaporative water loss in desert passerines reveals an expanding threat of lethal dehydration. Proceedings of the National Academy of Sciences 114: 2283–88. https://doi.org/10.1073/pnas.1613625114
Allison, E.H., A.L. Perry, M.-C. Badjeck, et al. 2009. Vulnerability of national economies to the impacts of climate change on fisheries. Fish and Fisheries 10: 173–96. https://doi.org/10.1111/j.1467-2979.2008.00310.x
Arrhenius, S. 1896. On the influence of carbonic acid in the air upon the temperature of the Earth. Philosophical Magazine and Journal of Science 41: 237–76. https://doi.org/10.1080/14786449608620846
Barbet-Massin, M., B.A. Walther, W. Thuiller, et al. 2009. Potential impacts of climate change on the winter distribution of Afro-Palaearctic migrant passerines. Biology Letters 5: 248–51. https://doi.org/10.1098/rsbl.2008.0715
Battarbee, R.W. 2014. The rediscovery of the Aldabra banded snail, Rhachistia aldabrae. Biology Letters 10: 20140771. https://doi.org/10.1098/rsbl.2014.0771
Bazelet, C., and P. Naskrecki. 2014. Pseudosaga maraisi. The IUCN Red List of Threatened Species 2014: e.T62452865A62452868. http://doi.org/10.2305/IUCN.UK.2014-3.RLTS.T62 452865A62452868.en
Beale, C.M., N.E. Baker, M.J. Brewer, et al. 2013. Protected area networks and savannah bird biodiversity in the face of climate change and land degradation. Ecology letters 16: 1061–68. https://doi.org/10.1111/ele.12139
BirdLife International. 2016. Alauda razae. The IUCN Red List of Threatened Species 2016: e.T22717428A94531580. http://doi.org/10.2305/IUCN.UK.2018-2.RLTS.T22717428A131103086.en
Boko, M., I. Niang, A. Nyong, et al. 2007. Africa. In: Climate Change 2007: Impacts, Adaptation and Vulnerability, ed. by S. Solomon et al. (Cambridge: Cambridge University Press). https://www.ipcc.ch/site/assets/uploads/2018/02/ar4-wg2-chapter9-1.pdf
Both, C., S. Bouwhuis, C.M. Lessells, et al. 2006. Climate change and population declines in a long-distance migratory bird. Nature 441: 81–83. https://doi.org/10.1038/nature04539
Branch, T.A., B.M. DeJoseph, L.J. Ray, et al. 2013. Impacts of ocean acidification on marine seafood. Trends in Ecology and Evolution 28: 178–86. https://doi.org/10.1016/j.tree.2012.10.001
Burton, M.E.H., J.R. Poulsen, M.E. Lee, et al. 2017. Reducing carbon emissions from forest conversion for oil palm agriculture in Gabon. Conservation Letters 10: 297–307. https://doi.org/10.1111/conl.12265
Carolin, S.A., R.T. Walker, C.C. Day, et al. 2019. Precise timing of abrupt increase in dust activity in the Middle East coincident with 4.2 ka social change. Proceedings of the National Academy of Sciences 116: 67–72. https://doi.org/10.1073/pnas.1808103115
Carrington, D. Why the Guardian is changing the language it uses about the environment. Guardian. https://gu.com/p/bfgxm
Carr, J.A., A.F. Hughes, and W.B. Foden. 2014. A climate change vulnerability assessment of West African species. Technical Report (Cambridge: UNEP-WCMC). http://parcc.protectedplanet.net/assets/IUCN_species_vulnerability-181b4593dd469dcba033b1f06aaa3cd7c7678424c3a2b056578c9582bd5bf7fb.pdf
Chauka, L.J. 2016. Tanzanian reef building corals may succumb to bleaching events: Evidences from coral-symbiodinium symbioses. In: Estuaries: A Lifeline of Ecosystem Services in the Western Indian Ocean, ed. by S. Diop et al. (Cham: Springer). https://doi.org/10.1007/978-3-319-25370-1
Conradie, S.R., S.M. Woodbourne, S.J. Cunningham, et al. 2019. Chronic, sublethal effects of high temperatures will cause severe declines in southern African arid-zone birds during the 21st Century. Proceedings of the National Academy of Sciences 116: in press.
Craig, M.H., R.W. Snow, and D. le Sueur. 1999. A climate-based distribution model of malaria transmission in sub-Saharan Africa. Parasitology Today 15: 105–11. https://doi.org/10.1016/S0169-4758(99)01396-4
Craparo, A.C.W., P.J.A. van Asten, P. Läderach, et al. 2015. Coffea arabica yields decline in Tanzania due to climate change: Global implications. Agricultural and Forest Meteorology 207: 1–10. https://doi.org/10.1016/j.agrformet.2015.03.005
Crump, M.L., F.R. Hensley, and K.L. Clark, 1992. Apparent decline of the golden toad: Underground or extinct? Copia 1992: 413–20.
Cunningham, S.J., R.O. Martin, C.L. Hojem, et al. 2013. Temperatures in excess of critical thresholds threaten nestling growth and survival in a rapidly-warming arid savanna: A study of common fiscals. PLoS ONE 8: e74613. https://doi.org/10.1371/journal.pone.0074613
DeMenocal, P.B. 2001. Cultural responses to climate change during the late Holocene. Science 292: 667–73. https://doi.org/10.1126/science.1059287
Dietz, S., A. Bowen, C. Dixon, et al. 2016. ‘Climate value at risk’ of global financial assets. Nature Climate Change 6: 676–79. https://doi.org/10.1038/nclimate2972
Dimitrov, D., D. Nogués-Bravo, and N. Scharff. 2012. Why do tropical mountains support exceptionally high biodiversity? The Eastern Arc Mountains and the drivers of Saintpaulia diversity. PloS ONE 7: e48908. https://doi.org/10.1371/journal.pone.0048908
du Plessis, K.L., R.O. Martin, P.A.R. Hockey, et al. 2012. The costs of keeping cool in a warming world: Implications of high temperatures for foraging, thermoregulation and body condition of an arid-zone bird. Global Change Biology 18: 2063–3070. https://doi.org/10.1111/j.1365-2486.2012.02778.x
Engelbrecht, F.A., J.L. McGregor, and C.J. Engelbrecht. 2009. Dynamics of the Conformal‐Cubic Atmospheric Model projected climate‐change signal over southern Africa. International Journal of Climatology 29: 1013–33. https://doi.org/10.1002/joc.1742
Fagotto, M., and M. Gattoni. 2016. West Africa is being swallowed by the sea. Foreign Policy. http://atfp.co/2tUZCaM
Fitchett, J.M., and S.W. Grab. 2014. A 66‐year tropical cyclone record for south‐east Africa: Temporal trends in a global context. International Journal of Climatology 34: 3604–15. https://doi.org/10.1002/joc.3932
Flörke, M., C. Schneider, and R.I. McDonald. 2018. Water competition between cities and agriculture driven by climate change and urban growth. Nature Sustainability 1: 51–58. https://doi.org/10.1038/s41893-017-0006-8
Foden, W., G.F. Midgley, G. Hughes, et al. 2007. A changing climate is eroding the geographical range of the Namib Desert tree Aloe through population declines and dispersal lags. Diversity and Distributions 13: 645–53. https://doi.org/10.1111/j.1472-4642.2007.00391.x
Fordham, D.A., C. Bertelsmeier, B.W. Brook, et al. 2018. How complex should models be? Comparing correlative and mechanistic range dynamics models. Global Change Biology 24: 1357–70. https://doi.org/10.1111/gcb.13935
Forster, P., V. Ramaswamy, P. Artaxo, et al. 2007. Changes in atmospheric constituents and in radiative forcing. In: Climate Change 2007: The Physical Science Basis, ed. by S. Solomon et al. (Cambridge: Cambridge University Press). https://www.ipcc.ch/site/assets/uploads/2018/02/ar4-wg1-chapter2-1.pdf
Garpe, K.C., S.A.S. Yahya, U. Lindahl, et al. 2006. Long-term effects of the 1998 coral bleaching event on reef fish assemblages. Marine Ecology Progress Series 315: 237–47. https://doi.org/10.3354/meps315237
Gillis, J. 2017. Earth sets a temperature record for the third straight year. New York Times. https://nyti.ms/2jAdWlA
Gonedelé B.S., I. Koné, A.E., Bitty, et al. 2012. Distribution and conservation status of catarrhine primates in Côte d’Ivoire (West Africa). Folia Primatologica 83: 11–23. https://doi.org/10.1159/000338752
Grab, S., and A. Craparo. 2011. Advance of apple and pear tree full bloom dates in response to climate change in the southwestern Cape, South Africa: 1973–2009. Agricultural and Forest Meteorology 151: 406–13. http://doi.org/10.1016/j.agrformet.2010.11.001
Gynther, I., N. Waller, and L.K.-P. Leung. 2016. Confirmation of the extinction of the Bramble Cay melomys Melomys rubicola on Bramble Cay, Torres Strait (Brisbane: EHP). https://environment.des.qld.gov.au/wildlife/threatened-species/documents/bramble-cay-melomys-survey-report.pdf
Harris, N.L., S. Brown, S.C. Hagen, et al. 2012. Baseline map of carbon emissions from deforestation in tropical regions. Science 336: 1573–76. https://doi.org/10.1126/science.1217962
Hole, D.G., S.G. Willis, D.J. Pain, et al. 2009. Projected impacts of climate change on a continent-wide protected area network. Ecology Letters 12: 420–31. https://doi.org/10.1111/j.1461-0248.2009.01297.x
Houniet, D.T., W. Thuiller, and K.A. Tolley. 2009. Potential effects of predicted climate change on the endemic South African Dwarf Chameleons, Bradypodion. African Journal of Herpetology 58: 28–35. https://doi.org/10.1080/21564574.2009.9635577
Hsiang, S.M., and A.H. Sobel. 2016. Potentially extreme population displacement and concentration in the tropics under non-extreme warming. Scientific Reports 6: 25697. https://doi.org/10.1038/srep25697
Huntley, B., and P. Barnard. 2012. Potential impacts of climatic change on southern African birds of fynbos and grassland biodiversity hotspots. Diversity and Distributions 18: 1–13. https://doi.org/10.1111/j.1472-4642.2012.00890.x
IPCC. 2014: Climate Change 2014: Synthesis Report (Geneva: IPCC). https://www.ipcc.ch/report/ar5/syr
Ito, T., S. Minobe, M.C. Long, et al. 2017. Upper ocean O2 trends: 1958–2015. Geophysical Research Letters 44: 4214–23. https://doi.org/10.1002/2017GL073613
Jackson, R.B., C. Le Quéré, R.M. Andrew, et al. 2018. Global energy growth is outpacing decarbonization. Environmental Research Letters 13: 120401. https://doi.org/10.1088/1748-9326/aaf303
Jaramillo J., E. Muchugu, F.E. Vega, et al. 2011. Some like it hot: The influence and implications of climate change on coffee berry borer (Hypothenemus hampei) and coffee production in East Africa. PLoS ONE 6: e24528. https://doi.org/10.1371/journal.pone.0024528
Jezkova, T., and J.J. Wiens. 2016. Rates of change in climatic niches in plant and animal populations are much slower than projected climate change. Proceedings of the Royal Society B 283: 20162104. https://doi.org/10.1098/rspb.2016.2104
Jolly, W.M., M.A. Cochrane, P.H. Freeborn, et al. 2015. Climate-induced variations in global wildfire danger from 1979 to 2013. Nature Communications 6: 8537. https://doi.org/10.1038/ncomms8537
Kaempffert, W. 1956. Warmer climate on the Earth may be due to more carbon dioxide in the air. New York Times. https://nyti.ms/2zYC2Ot
Kaniewski, D., E. van Campo, J. Guiot, et al. 2013. Environmental roots of the Late Bronze Age crisis. PLoS ONE 8: e71004. https://doi.org/10.1371/journal.pone.0071004
Khatiwala, S., F. Primeau, and T. Hall. 2009. Reconstruction of the history of anthropogenic CO2 concentrations in the ocean. Nature 462: 346–49. https://doi.org/10.1038/nature08526
Knouft, J.H., and D.L. Ficklin. 2017. The potential impacts of climate change on biodiversity in flowing freshwater systems. Annual Review of Ecology, Evolution, and Systematics 48: 111–33. https://doi.org/10.1146/annurev-ecolsys-110316-022803
Koh, L.P., R.R. Dunn, N.S. Sodhi, et al. 2004. Species coextinctions and the biodiversity crisis. Science 305: 1632–34. https://doi.org/10.1126/science.1101101
Kreyling, J., D. Wana, and C. Beierkuhnlein. 2010. Potential consequences of climate warming for tropical plant species in high mountains of southern Ethiopia. Diversity and Distributions 16: 593–605. https://doi.org/10.1111/j.1472-4642.2010.00675.x
La Sorte, F.A., S.H.M. Butchart, W. Jetz, et al. 2014. Range-wide latitudinal and elevational temperature gradients for the world’s terrestrial birds: Implications under global climate change. PLoS ONE 9: e98361. https://doi.org/10.1371/journal.pone.0098361
Laloë, J.-O., J. Cozens, B. Renom, et al. 2014. Effects of rising temperature on the viability of an important sea turtle rookery. Nature Climate Change 4: 513–18. https://doi.org/10.1038/nclimate2236
Lam, V.W.Y., W.W.L. Cheung, W. Swartz, et al. 2012. Climate change impacts on fisheries in West Africa: Implications for economic, food and nutritional security. African Journal of Marine Science 34: 103–17. http://doi.org/10.2989/1814232X.2012.673294
Le Quéré, C.L., R.M. Andrew, P. Friedlingstein, et al. 2018. Global carbon budget 2018. Earth System Science Data 10: 2141–94. https://doi.org/10.5194/essd-10-2141-2018
Leduc, A.O.H.C., P.L. Munday, G.E. Brown, et al. 2013. Effects of acidification on olfactory-mediated behaviour in freshwater and marine ecosystems: A synthesis. Philosophical Transactions of the Royal Society B 368: 20120447. http://doi.org/10.1098/rstb.2012.0447
Leslie, A.J., and J.R. Spotila. 2001. Alien plant threatens Nile crocodile (Crocodylus niloticus) breeding in Lake St. Lucia, South Africa. Biological Conservation 98: 347–55. https://doi.org/10.1016/S0006-3207(00)00177-4
Linder, J.M. 2013. African primate biodiversity threatened by “new wave” of industrial oil palm expansion. African Primates 8: 25–38.
Linder, J.M., and R.E. Palkovitz. 2016. The threat of industrial oil palm expansion to primates and their habitats. In: Ethnoprimatology, ed. by M. Waller (Cham: Springer). https://doi.org/10.1007/978-3-319-30469-4
Long, M.C., C. Deutsch, and T. Ito. 2016. Finding forced trends in oceanic oxygen. Global Biogeochemical Cycles 30: 381–97. https://doi.org/10.1002/2015GB005310
Maxwell, D., N. Majid, H. Stobaugh, et al. 2014. Lessons learned from the Somalia famine and the greater Horn of Africa crisis 2011–2012 (Medford: Feinstein International Center, Tufts University). http://fic.tufts.edu/publication-item/famine-somalia-crisis-2011-2012
McClanahan, T.R., M. Ateweberhan, C.A. Muhando, et al. 2007. Effects of climate and seawater temperature variation on coral bleaching and mortality. Ecological Monographs 77: 503–25. https://doi.org/10.1890/06-1182.1
McClean, C.J., N. Doswald, W. Küper, et al. 2006. Potential impacts of climate change on Sub-Saharan African plant priority area selection. Diversity and Distributions 12: 645–55. https://doi.org/10.1111/j.1472-4642.2006.00290.x
McKechnie, A.E., and B.O. Wolf. 2010. Climate change increases the likelihood of catastrophic avian mortality events during extreme heat waves. Biology Letters 6: 253–56. https://doi.org/10.1098/rsbl.2009.0702
Medek, D.E., J. Schwartz, and S.S. Myers. 2017. Estimated effects of future atmospheric CO2 concentrations on protein intake and the risk of protein deficiency by country and region. Environmental Health Perspectives 125: 087002. https://doi.org/10.1289/EHP41
Mekasha, A., L. Nigatu, K. Tesfaye, et al. 2013. Modeling the response of tropical highland herbaceous grassland species to climate change: The case of the Arsi Mountains of Ethiopia. Biological Conservation 168: 169–75. https://doi.org/10.1016/j.biocon.2013.09.020
Merone, L, and P. Tait. 2018. ‘Climate refugees’: Is it time to legally acknowledge those displaced by climate disruption? Australian and New Zealand Journal of Public Health 6: 508–09. https://doi.org/10.1111/1753-6405.12849
Milne, R., S.J. Cunningham, A.T. Lee, et al. 2015. The role of thermal physiology in recent declines of birds in a biodiversity hotspot. Conservation Physiology 3: p.cov048. https://doi.org/10.1093/conphys/cov048
Mollica, N.R., W. Guo, A.L. Cohen, et al. 2018. Ocean acidification affects coral growth by reducing skeletal density. Proceedings of the National Academy of Sciences 115: 1754–59. https://doi.org/10.1073/pnas.1712806115
Myers, S.S., A. Zanobetti, I. Kloog, et al. 2014. Increasing CO2 threatens human nutrition. Nature 510: 139–42. https://doi.org/10.1038/nature13179
NASA. 2018. Forcings in GISS Climate Model: Historical Data. https://data.giss.nasa.gov/modelforce/ghgases
NOAA. 2016. Extended Reconstructed Sea Surface Temperature (ERSST), v. 4. http://doi.org/10.7289/V5KD1VVF
NOAA. 2018a. Climate at a Glance: Global Time Series, December 2018. https://www.ncdc.noaa.gov/cag
NOAA. 2018b. NOAA Earth System Research Laboratory: Global Monitoring Division, December 2018. https://www.esrl.noaa.gov/gmd/ccgg/trends
NOAA. 2018c. State of the Climate: Global Climate Report for April 2018. https://www.ncdc.noaa.gov/sotc/global/201804
O’Connor, T.G., and G.A. Kiker. 2004. Collapse of the Mapungubwe society: Vulnerability of pastoralism to increasing aridity. Climatic Change 66: 49–66. https://doi.org/10.1023/B:CLIM.0000043192.19088.9d
O’Reilly, C.M., S. Sharma, D.K. Gray, et al. 2015. Rapid and highly variable warming of lake surface waters around the globe. Geophysical Research Letters 42: 10773–81. https://doi.org/10.1002/2015GL066235
O’Reilly, C.M., S.R. Alin, P.-D. Plisnier, et al. 2003. Climate change decreases aquatic ecosystem productivity of Lake Tanganyika, Africa. Nature 424: 766–68. https://doi.org/10.1038/nature01833
Ordway, E.M., R.L. Naylor, R.N. Nkongho, et al. 2019. Oil palm expansion and deforestation in Southwest Cameroon associated with proliferation of informal mills. Nature Communications 10: 114. https://doi.org/10.1038/s41467-018-07915-2
Pinsky, M.L., A.M. Eikeset, D.J. McCauley, et al., 2019. Greater vulnerability to warming of marine versus terrestrial ectotherms. Nature 569: 108–11. https://doi.org/10.1038/s41586-019-1132-4
Pollom, R. 2017. Hippocampus capensis. The IUCN Red List of Threatened Species 2017: e.T10056A54903534. http://doi.org/10.2305/IUCN.UK.2017-3.RLTS.T10056A54903534.en
Ponce-Reyes, R., A.J. Plumptre, D. Segan, et al. 2017. Forecasting ecosystem responses to climate change across Africa’s Albertine Rift. Biological Conservation 209: 464–72. https://doi.org/10.1016/j.biocon.2017.03.015
Reizenberg, J.-L., L.E. Bloy, O.L.F. Weyl, et al. 2019. Variation in thermal tolerances of native freshwater fishes in South Africa’s Cape Fold Ecoregion: Examining the east-west gradient in species’ sensitivity to climate warming. Journal of Fish Biology 94: 103–12. https://doi.org/10.1111/jfb.13866
Renner, S.S., and C.M. Zohner. 2018. Climate change and phenological mismatch in trophic interactions among plants, insects, and vertebrates. Annual Review of Ecology, Evolution, and Systematics 49: 165–82. https://doi.org/10.1146/annurev-ecolsys-110617-062535
Rey, B., A. Fuller, D. Mitchell, et al. 2017. Drought-induced starvation of aardvarks in the Kalahari: An indirect effect of climate change. Biology Letters 13: 20170301. https://doi.org/10.1098/rsbl.2017.0301
Roggatz, C.C., M. Lorch, J.D. Hardege, et al. 2016. Ocean acidification affects marine chemical communication by changing structure and function of peptide signalling molecules. Global Change Biology 22: 3914–26. https://doi.org/10.1111/gcb.13354
Russo, S., A.F. Marchese, J. Sillmann, et al. 2016. When will unusual heat waves become normal in a warming Africa? Environmental Research Letters 11: 054016. https://doi.org/10.1088/1748-9326/11/5/054016
Samways, M.J. 2005. Breakdown of butterflyfish (Chaetodontidae) territories associated with the onset of a mass coral bleaching event. Aquatic Conservation 15: S101–S107. https://doi.org/10.1002/aqc.694
Serdeczny, O., S. Adams, F. Baarsch, et al. 2017. Climate change impacts in Sub-Saharan Africa: From physical changes to their social repercussions. Regional Environmental Change 17: 1585–600. https://doi.org/10.1007/s10113-015-0910-2
Simmons, R.E., P. Barnard, W.R.J. Dean, et al. 2004. Climate change and birds: Perspectives and prospects from southern Africa. Ostrich 75: 295–308. https://doi.org/10.2989/00306520409485458
Sinervo, B., F. Mendez-De-La-Cruz, D.B. Miles, et al. 2010. Erosion of lizard diversity by climate change and altered thermal niches. Science 328: 894–99. https://doi.org/10.1126/science.1184695
Siraj, A.S., M. Santos-Vega, M.J. Bouma, et al. 2014. Altitudinal changes in malaria incidence in highlands of Ethiopia and Colombia. Science 343: 1154–58. https://doi.org/10.1126/science.1244325
Smith, A., M.C. Schoeman, M. Keith, et al. 2016. Synergistic effects of climate and land-use change on representation of African bats in priority conservation areas. Ecological Indicators 69: 276–83. http://doi.org/10.1016/j.ecolind.2016.04.039
Storlazzi, C.D., S.B. Gingerich, A. van Dongeren, et al. 2018. Most atolls will be uninhabitable by the mid-21st century because of sea-level rise exacerbating wave-driven flooding. Science Advances 4: eaap9741. https://doi.org/10.1126/sciadv.aap9741
Strydom, S., and M.J. Savage. 2016. A spatio-temporal analysis of fires in South Africa. South African Journal of Science 112: 1–8. https://doi.org/10.17159/sajs.2016/20150489
Thieme, M.L., B. Lehner, R. Abell, et al. 2010. Exposure of Africa’s freshwater biodiversity to a changing climate. Conservation Letters 3:324–31. https://doi.org/10.1111/j.1755-263X.2010.00120.x
Thomas, C.D., A. Cameron, R.E. Green, et al. 2004. Extinction risk from climate change. Nature 427: 145–48. https://doi.org/10.1038/nature02121
Tuqa, J.H., P. Funston, C. Musyoki, et al. 2014. Impact of severe climate variability on lion home range and movement patterns in the Amboseli ecosystem, Kenya. Global Ecology and Conservation 2: 1–10. https://doi.org/10.1016/j.gecco.2014.07.006
Uhe, P., S. Philip, S. Kew, et al. 2017. Kenya drought, 2016. https://wwa.climatecentral.org/analyses/kenya-drought-2016
Valenzuela, N., and V. Lance. 2004. Temperature-Dependent Sex Determination in Vertebrates (Washington: Smithsonian Books). https://doi.org/10.5479/si.9781944466213
van Vliet, M.T., D. Ludwig, and P. Kabat. 2013. Global streamflow and thermal habitats of freshwater fishes under climate change. Climate Change 121: 739–54. https://doi.org/10.1007/s10584-013-0976-0
van Wilgen, N.J., V. Goodall, S. Holness, et al. 2016. Rising temperatures and changing rainfall patterns in South Africa’s national parks. International Journal of Climatology 36: 706–21. https://doi.org/10.1002/joc.4377
Vickery, J.A., S.R. Ewing, K.W. Smith, et al. 2014. The decline of Afro‐Palaearctic migrants and an assessment of potential causes. Ibis 156: 1–22. https://doi.org/10.1111/ibi.12118
Vijay, V., S.L. Pimm, C.N. Jenkins, et al. 2016. The impacts of oil palm on recent deforestation and biodiversity loss. PloS ONE 11: e0159668. https://doi.org/10.1371/journal.pone.0159668
Vittor, A.Y., R.H. Gilman, J. Tielsch, et al. 2006. The effect of deforestation on the human-biting rate of Anopheles darlingi, the primary vector of falciparum malaria in the Peruvian Amazon. American Journal of Tropical Medicine and Hygiene 74: 3–11. https://doi.org/10.4269/ajtmh.2006.74.3
Wang, X., F. Chen, J. Zhang, et al. 2010. Climate, desertification, and the rise and collapse of China’s historical dynasties. Human Ecology 38: 157–72. https://doi.org/10.1007/s10745-009-9298-2
Warren, R., J. Price, J. VanDerWal, et al. 2018. The implications of the United Nations Paris Agreement on climate change for globally significant biodiversity areas. Climatic Change 147: 395–409. https://doi.org/10.1007/s10584-018-2158-6
Watts, N., W.N. Adger, S. Ayeb-Karlsson, et al. 2017. The Lancet Countdown: Tracking progress on health and climate change. Lancet 389: 1151–64. https://doi.org/10.1016/S0140-6736(16)32124-9
Weiss, H., and R.S. Bradley. 2001. What drives societal collapse? Science 291: 609–10. https://doi.org/10.1126/science.1058775
Whitehead, P., R. Wilby, R. Battarbee, et al. 2009. A review of the potential impacts of climate change on surface water quality. Hydrological Sciences Journal 54:101–23. https://doi.org/10.1623/hysj.54.1.101
Whittington-Jones, G.M., R.T.F. Bernard, and D.M. Parker. 2011. Aardvark burrows: A potential resource for animals in arid and semi-arid environments. African Zoology 46: 362–70. https://doi.org/10.3377/004.046.0215
Wiens, J.J. 2016. Climate-related local extinctions are already widespread among plant and animal species. PLoS Biology 14: e2001104. https://doi.org/10.1371/journal.pbio.2001104
Wiley, E.M., and A.R. Ridley. 2016. The effects of temperature on offspring provisioning in a cooperative breeder. Animal Behaviour 117: 187–95. https://doi.org/10.1016/j.anbehav.2016.05.009
Williams, J.W., S.T. Jackson, and J.E. Kutzbach. 2007. Projected distributions of novel and disappearing climates by 2100 AD. Proceedings of the National Academy of Sciences 104: 5738–42. https://doi.org/10.1073/pnas.0606292104
WRI (World Resources Institute). 2019 Climate analysis indicators tool: WRI’s climate data explorer. http://cait2.wri.org
Zabel, F., B. Putzenlechner, and W. Mauser. 2014. Global agricultural land resources—a high resolution suitability evaluation and its perspectives until 2100 under climate change conditions. PLoS ONE 9: e107522. https://doi.org/10.1371/journal.pone.0107522
Zietsman, J., L.L. Dreyer, and K.J. Esler. 2008. Reproductive biology and ecology of selected rare and endangered Oxalis L. (Oxalidaceae) plant species. Biological Conservation 141: 1475–83. http://doi.org/10.1016/j.biocon.2008.03.017