8. Extinction Is Forever
© 2019 J.W. Wilson and R.B. Primack, CC BY 4.0 https://doi.org/10.11647/OBP.0177.08
8.1 What is Extinction? p. 259
8.2 Rates of Extinction p. 259
8.3 When is a Species Extinct? p. 260
8.4 History of Extinctions in Sub-Saharan Africa p. 263
8.5 Which Species are at Risk of Extinction? p. 271
8.6 Characteristics of Threatened Species p. 274
8.7 Problems of Small Populations p. 277
8.8 Is De-extinction a Solution? p. 285
8.10 Topics for Discussion p. 289
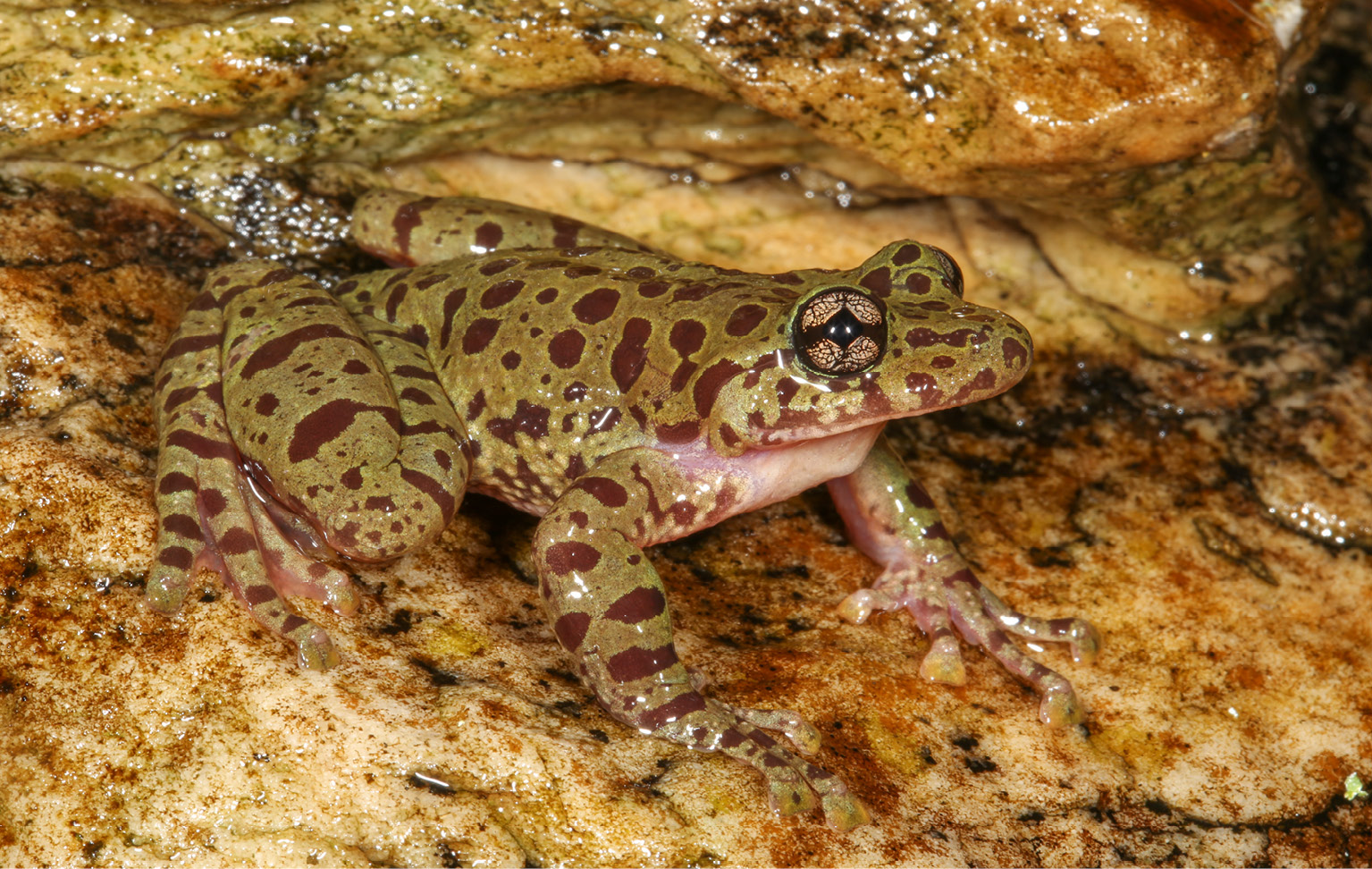
Extinctions are seldom attributable to only one threat; rather, multiple stressors may act synergistically to drive the demise of a species. Pictured here is a Hewitt’s ghost frog (Heleophryne hewetti, EN), which is globally restricted to an area of 140 km2 in the Cape Floristic Region. It is threatened by alien vegetation, overly frequent fires, erosion, siltation, and construction of roads and reservoirs, all of which deteriorate its clear, fast-flowing stream habitat. Photograph by Werner Conradie, CC BY 4.0.
Species have evolved and disappeared since the very first species (thought to be microorganisms living in hydrothermal vents) made an appearance on Earth. Some species outcompeted others for access to limiting resources; some were driven to extinction by dangerous pathogens; some just found it hard to survive in constantly evolving ecosystems. While many extinction events have been rather limited in scope and, hence, caused only one or a few extinctions at a time, there have been instances where perturbations were so impactful that they drove very large numbers of species to extinction over a short period of time. There have been five such past mass extinction events—periods marked by the sudden and dramatic loss of a large percentage of species (Figure 8.1). But these mass extinctions have also been followed by periods that favoured increased rates of speciation, during which new species evolved to fill the niches left empty by the extinctions.
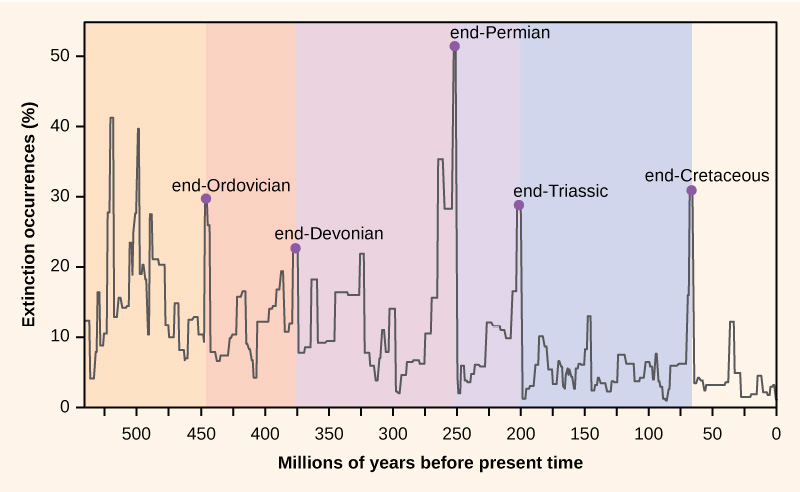
Figure 8.1 There have been five past mass extinction events—periods when natural events changed Earth’s environment so dramatically that between 60–95% of species were wiped away forever—over Earth’s geological history. So far, the most dramatic extinction event occurred at the end of the Permian period, about 250 million years ago, and thought to be the result of widespread volcanic activity and climate change. The most recent mass extinction, at the end of the Cretaceous period about 65 million years ago and thought to be the result of a massive asteroid impact, saw the disappearance of non-avian dinosaurs. Source: OpenStax, 2019, CC BY 4.0.
Nature’s ability to balance extinctions with speciation was greatly disturbed around 300,000 years ago, when Homo sapiens made their appearance on Earth. Since then, humans have gradually increased their dominance on the natural world, leading to large-scale restructuring and destruction of biological communities. Human modifications of Earth’s climatic, biological, and geochemical environments accelerated greatly during the rise of agriculture (12,000–15,000 years ago) and again during the Industrial Revolution (1760–1840), when fossil fuel usage and urbanisation became the norm. Now, many scientists recognise today’s new and distinct human-dominated geological epoch, the Anthropocene (Waters et al., 2015). One notable feature of the Anthropocene is that species extinctions are increasing at such rapid rates that many conservation biologists now recognise that we are also witnessing the beginnings of Earth’s sixth extinction episode (Barnosky et al., 2011; Ceballos et al., 2017). However, unlike previously, this extinction episode is caused by human activities rather than natural events.
8.1 What is Extinction?
The term “extinct” has several nuances in conservation biology, and its meaning can vary somewhat depending on the context:
- A species is globally extinct when no individuals of that species remains alive anywhere in the world. The bluebuck (Hippotragus leucophaeus, EX) has been globally Extinct since the last individual was shot around 1800 (Kerley et al., 2009).
- Four (possibly seven) species of cycad (Encephalartos spp.)—ancient seed plants that were dominant in the age of the dinosaurs—are currently considered extinct in the wild; in other words, they exist only in cultivation; in captivity; or another human-managed situation (IUCN, 2019).
- A species is locally extinct, also called extirpated, when it is extinct in a part of its historic range but can still be found elsewhere in the world. Cheetahs (Acinonyx jubatus, VU) once roamed throughout much of Africa, but are now extirpated in over 90% of their historical range (Durant et al., 2017).
- A species is ecologically extinct (also called functionally extinct) if it persists at such low numbers that its role in an ecosystem is negligible. Africa’s vultures are ecologically extinct over much of their range and thus unable to remove diseased carcasses from the environment, posing both an ecological and socio-economic hazard (see Box 4.4).
8.2 Rates of Extinction
If extinction and speciation are natural processes, an obvious question follows: “Why should we care about the loss of biodiversity?” The answer concerns not individual species extinctions as much as the increasing rate of these extinctions (Figure 8.2). While a species can be wiped off Earth over a relatively short period of time, speciation typically occurs slowly as the genetic makeup of a population shifts over thousands of years. Unfortunately, we are currently losing species 1,000 times faster than natural background extinction rates (for mammals estimated to be 1.8 extinctions per 10,000 species per 100 years, Barnosky et al., 2011), and future rates may be 10,000 times higher that background rates (de Vos et al., 2015). Because over 99% of current species extinctions have been linked to human activity rather than natural processes (Pimm et al., 2014), observations on past extinctions and subsequent speciation may not apply to the present. Moreover, unlike before, humans now share the planet with the species we are wiping out. These losses mean that we are also losing the benefits we gain from nature (Chapter 4) at unprecedented rates.
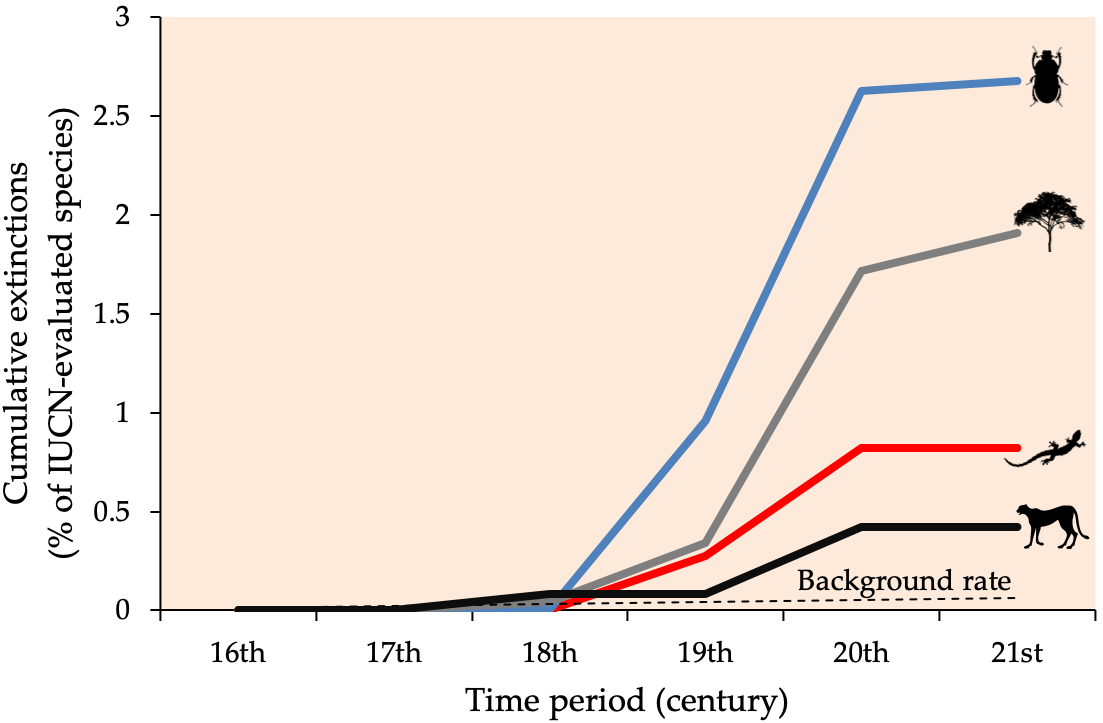
Figure 8.2 Percentage of Sub-Saharan Africa invertebrates, plants, reptiles, and mammals that have gone Extinct, Extinct in the Wild, and likely Extinct since the year 1500. Dashed line represents the natural rate of extinctions expected without human influences. After Ceballos et al., 2015, CC BY 4.0.
8.3 When is a Species Extinct?
While the term “extinction” is relatively easy to define (Section 8.1), determining whether a species is indeed extinct is a more difficult task. One of the most important questions conservation biologists grapple with is deciding how long to wait after the last observation before declaring a species extinct. Answering this question is particularly complicated when considering cryptic and shy species that are difficult to survey, sparsely distributed animals that are hard to find, or plants that are difficult to identify when not in flower.
To complicate matters, over the last few decades, biologists and their colleagues have rediscovered several species that were once thought to be extinct. These rediscovered species are often called Lazarus species, in reference to their apparent return to life. Recent examples include Burundi’s Bururi long-fingered frog (Cardioglossa cyaneospila. NT) rediscovered after a 60-year absence (Blackburn et al., 2016), a Tanzanian coral tree, (Erythrina schliebenii, CR) originally known from only one specimen collected from a deforested region in the 1930s (Clarke et al., 2011), and the coelacanth (Latimeria chalumnae, CR), a fish that was once thought to be extinct for millions of years (Balon et al., 1988). To avoid declaring more extant species as extinct, there is currently a practice of only declaring a species extinct after several decades of intensive searching and “there is no reasonable doubt that the last individual has died” (IUCN, 2012). Consequently, species, such as the black-spotted false shieldback katydid (Aroegas nigroornatus, CR) and the Ethiopian sedge, Cyperus chionocephalus, Critically Endangered, last seen in 1916 (Bazelet and Naskrecki, 2014) and 1836 (Contu, 2013) respectively, have not yet been declared extinct, even though the last individual may have died a long time ago. Similarly, as many as 15 African orchid species—a group that includes some of the most beautiful and specialised plants on Earth, some of which have not seen since 1890—are currently considered Critically Endangered but may actually be extinct (IUCN, 2019).
The rediscovery of species once thought to be extinct should not necessarily be considered a sign of conservation progress. In many cases, Lazarus species were simply overlooked because they were extremely rare and restricted to isolated locations. Such is the case for two forest birds from the island nation of São Tomé and Príncipe, namely the São Tomé grosbeak (Crithagra concolor, CR) and Newton’s fiscal (Lanius newtoni, CR). The grosbeak, the world’s largest canary (50% larger than the second largest canary), was for a long time known only from three specimens collected in 1888–1890; it was thus considered extinct, until its rediscovery over 100 years later, in 1991 (BirdLife International, 2018a). The fiscal shares a remarkably similar history: it was previously known only from records in 1888 and 1928, until its rediscovery in 1990 (BirdLife International, 2018b). Despite these rediscoveries, both species persist as very small (< 250 individuals) populations that are at risk from extinction due to ongoing habitat loss and the impact of invasive predators.
Because extinctions may not always happen immediately after a disturbance, conservation biologists must also consider the lag time between destructive human activities and eventual extinctions. This is illustrated in a study from Kenya’s Kakamega Forest, which found that only half of the species that will eventually go extinct due to habitat loss do so in the first 50 years following habitat fragmentation (Figure 8.3). Long-lived plants can have particularly long extinction lag times, sometimes of several centuries. For example, populations of the Saint Helena olive (Nesiota elliptica, EX) fell below viable levels in the mid-1800s, but the last individual died only in 2003, when the species was officially declared extinct (Cronk, 2016). Species that are doomed to eventual extinction are considered committed to extinction (also called functionally extinct), while the total number of species committed to extinction is referred to as an area’s extinction debt. In one study, researchers used the island biogeography theory to estimate that the average extinction debt for African forest primates was over 30%—that is, more than 30% of forest primates are predicted to go extinct because of habitat destruction and other human activities that have already happened (Cowlishaw, 1999).
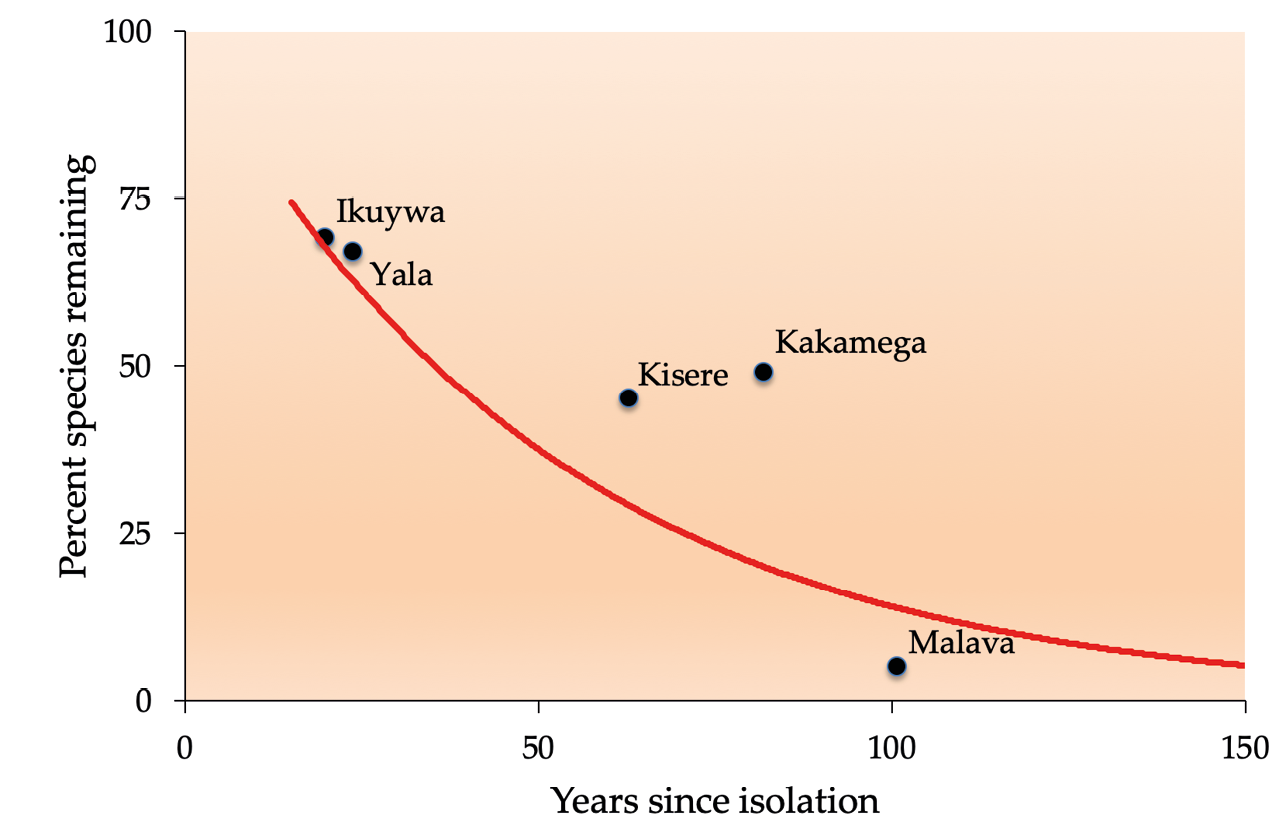
Figure 8.3 Percentage of bird species expected to persist over time in isolated forest patches in western Kenya. Because of extinction debt, not all species are expected to be extirpated immediately after fragmentation; instead there is a time lag between habitat loss and species losses. The image also illustrates how forest size and degree of isolation influences the speed of losses: Kakamega (the largest forest) loses species much slower than Malava, the smallest and most isolated forest. After Brooks et al., 1999, CC BY 4.0.
On a more positive note, extinction debts may also provide hope for conservation biologists, as the lingering presence of seriously imperilled species affords opportunities to prevent impending extinctions. Conservation biologists are currently illustrating how this can be done by preventing the extinction of three species of pale-coated, desert-adapted ungulates that were formerly common and widespread across the Sahel-Sahara region, namely the scimitar-horned oryx (Oryx dammah, EW), dama gazelle (Nanger dama, CR), and addax (Addax nasomaculatus, CR) (Durant et al., 2014; Brito et al., 2018; IUCN, 2019). The oryx once numbered around one million individuals, with herds of 10,000 seen as recently as 1936. But a population collapse soon followed: by 1985 only 500 oryx survived, and by 2000 it was declared Extinct in the Wild. The addax, relatively common as recently as in the 1970s, also experienced precipitous declines; today fewer than 30 individuals remain in the wild. Similarly, the once-common dama gazelle’s current global population numbers fewer than 250 individuals, fragmented among five subpopulations in Chad, Mali, and Niger. Conservationists noted initial declines already in the 1960s and 1970s, when wild individuals of all three species were caught to initiate captive breeding programmes. Luckily, all three species responded well to these programmes, and captive populations have grown so strong that reintroduction programmes (Section 11.2) have been initiated for the addax (in 1985, in Tunisia), dama gazelle (in 2015, in Morocco), and oryx (in 2016, in Chad). With several reintroductions seemingly successful, there is hope that viable populations of these iconic species may one day again roam free in their previous strongholds. This will only happen if we can reverse or mitigate the threats that causes their population collapses in the first place, namely uncontrolled and illegal hunting, as well as disturbances associated with agriculture, oil exploration, and inconsiderate drilling of wells for groundwater extraction.
8.4 History of Extinctions in Sub-Saharan Africa
Many people hold on to the romanticised belief that historical human societies lived in harmony with nature. Accumulated evidence however indicates that this is not true; early humans have caused extensive ecosystem changes and species extinctions since Homo sapiens appeared on Earth about 300,000 years ago. In fact, even before the arrival of humans, our ancestors had made a mark, by driving species to extinction as early as during the Pleistocene period, which started about 2.5 million years ago (Box 8.1). The impact of early humans was particularly devastating to the wildlife of North America, South America, and Australia, which saw the demise of nearly all their large (> 100 kg) mammals, most famously megaherbivores such as the mammoths (Mammuthus spp.). The Pleistocene extinctions were somewhat less devastating to wildlife in Africa, Europe, and Asia, possibly because large mammals on these continents evolved with human predators, allowing them to develop appropriate defence/escape mechanisms. Nevertheless, Africa’s wildlife did not completely escape the Pleistocene extinctions, as increasingly-sophisticated human activities during that time ensured the demise of as many as 28 large mammal groups, which included Africa’s sabre-toothed cats (Barbourofelidae), nearly all the elephant relatives (Proboscidae), as well as giant hartebeests (Megalotragus spp.), giant buffaloes (Pelorovis spp.), giant hyenas (Pachycrocuta spp.), and giant giraffes (Sivatherium spp.).
Box 8.1 Pleistocene Extinctions: Climate Change, Hominin Predation, or Both?
1FitzPatrick Institute of African Ornithology,
University of Cape Town, South Africa.
2Tropical Resource Ecology Programme, University of Zimbabwe,
Harare, Zimbabwe.
cummingdhm@gmail.com
Many scientists consider the present rapid loss of biodiversity to be the start of the 6th mass extinction following five previous extinction episodes (see Figure 8.1), each of which led to large-scale restructuring of Earth’s biodiversity. The 5th global mass extinction took place 65 million years ago (Ma) when a massive meteorite collided with the Earth, and resulted in the extinction of all non-avian dinosaurs, and much else besides. This 5th mass extinction event also marked the transition from the Cretaceous to the Tertiary epoch (65 to 2.5 Ma). The Tertiary epoch was followed by the Quaternary, which includes the Pleistocene period (2.5 million to ~ 12 thousand years ago) and more recently the Holocene period—marked by the development of agriculture, and the subsequent domination of the Earth’s resources by Homo sapiens. The Pleistocene is known for a mini mass-extinction of sorts, which saw the demise of species such as mammoths and sabre-tooth cats. However, unlike previous comprehensive mass extinctions, the Pleistocene was characterised by the extinction of mostly large mammals and very large island birds.
Attempts by scientists to explain these Pleistocene extinctions have been characterised by two centuries of controversy over whether they were caused by climate change or by predatory hominins—the evolutionary line of primates that gave rise to modern humans. The four main hypotheses advanced to account for the loss of Pleistocene fauna are: (i) climate change with minimal if any hominin influence (e.g. Faith et al., 2018); (ii) climate change together with some hominin influence (e.g. Barnosky et al., 2004); (iii) selective hominin predation aided by climate change (e.g. Bartlett et al., 2015), and (iv) hominin predation helped by other large predators without the influence of climate change (e.g. Janzen, 1983, Ripple and Van Valkenburgh, 2010).
The very close relationship between the dispersal of hominins out of Africa, the timing of their arrival elsewhere in the world, and the subsequent extinction of large mammals and birds, provided the primary (if challenged) evidence for human agency in non-African Late- Pleistocene extinctions (e.g. Surovell et al., 2005; Johnson, 2009; Ripple and Van Valkenburgh, 2010). As Haynes (2018) has remarked, “…the proponents of climate change as the only cause of the Late Pleistocene extinctions have not clearly explained how or why so many of the extinct megafaunal genera had survived numerous earlier climate changes.”
Similarly, Faith et al. (2018) have stated that the failure of Pleistocene megaherbivores to adapt to the emergence of C4 grasses was the primary driver of their extinction. However, this claim ignores evidence that many extinct herbivore genera and species previously survived changes in diet over time (Ripple and Van Valkenburgh, 2010), that the diets of particular species were known to vary with location (Ferranec, 2004), and that many large species, which are typically highly mobile generalists, would have had little trouble adapting their ranges and diets to changing climates.
Research and debate on Pleistocene extinctions have tended to focus on the demise of non-African large mammals in the Late Pleistocene, which coincided with the period when hominins (Homo erectus and later also H. sapiens) dispersed across the globe starting at about 2 Ma. In Africa, however, earliest hominins appeared some 7 Ma. It didn’t take long for these early African hominins to develop the skills necessary to manipulate the environment to their advantage. Setting the pace were the Australopithecines, who used stone tools to butcher mammalian carcasses between 4–3 Ma. The Australopithecines and the rest of a diverse group of large predators were joined by H. erectus at the beginning of the Pleistocene, about 2 Ma. This new, qualitatively unique, hunter was able to hunt collaboratively in bands, and was anatomically adapted to throw projectiles forcefully and accurately at large prey (Lombardo and Deaner, 2018). A large brain also placed high nutritional and energetic demands on H. erectus, that could best be met by obtaining meat and bone marrow from proboscidians, the elephant relatives (Surovell et al., 2005; Boschian et al., 2019). Early African hominins were thus well adapted to hunt large prey and contribute to the demise of wildlife, particularly megaherbivores (those over 1,000 kg), through the Early and Middle Pleistocene (Figure 8.A). Other large carnivores at the time may very well have helped hominins drive many Pleistocene herbivores to extinction (Janzen, 1983, Ripple and Van Valkenburgh, 2010; Van Valkenburgh et al., 2016). But this combination may ironically also have led to demise of many of the large Pleistocene predators, through co-extinctions, after their main prey base disappeared (Werdelin and Lewis, 2013).
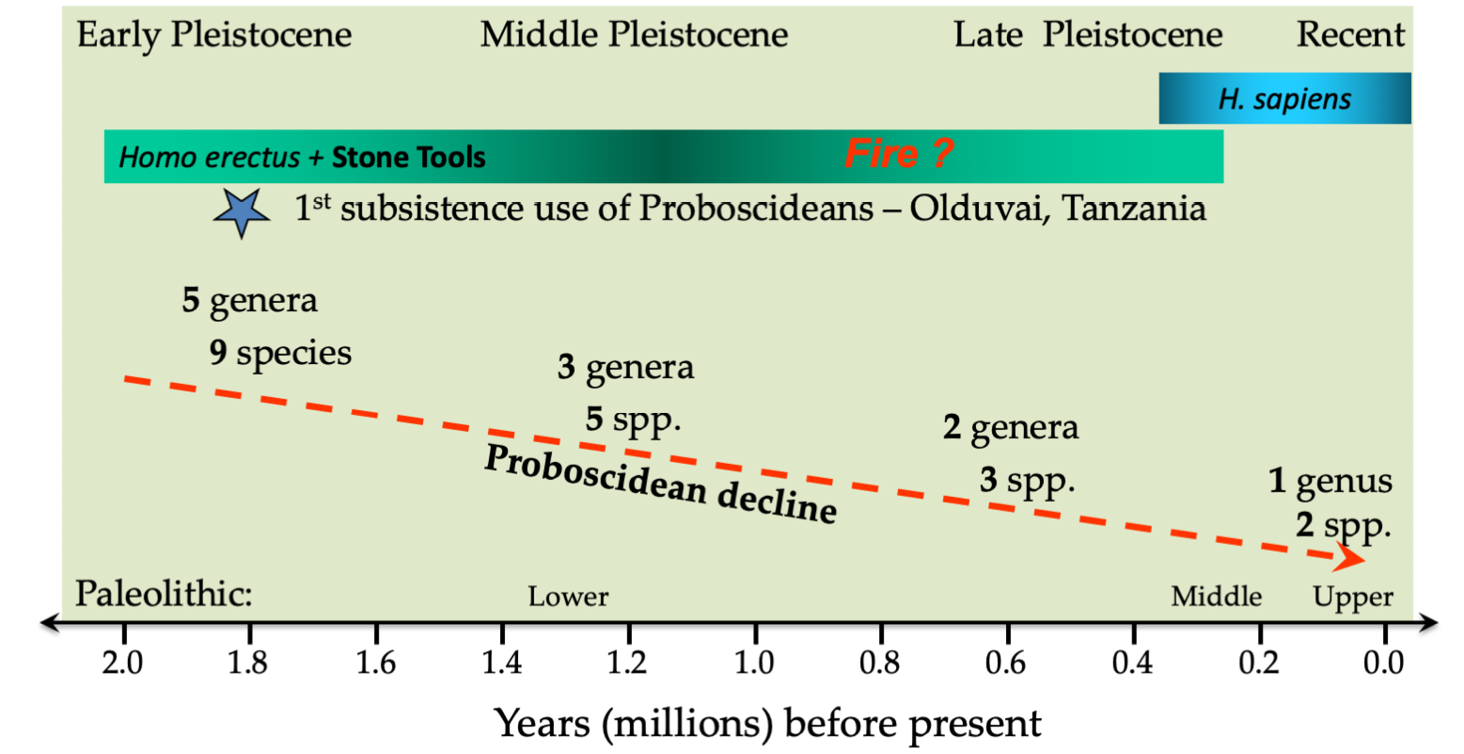
Figure 8.A Decline in African proboscidian (i.e. elephant relatives) diversity through the Early, Middle and Late Pleistocene in relation to the emergence of Homo erectus and H. sapiens. Similar patterns also occurred in the extinction of large carnivores and giant pigs/hogs. Source: Cumming, 2007, CC BY 4.0.
It thus seems likely that the emergence of a novel and increasingly effective predator during the Early Pleistocene, rather than climate change, was the ultimate factor that tipped the balance against the iconic species that disappeared soon after hominins appeared on Earth. It is worth noting that there is a clear relationship between body size and extinction risk (Figure 8.B), the result of large animals’ relatively long generation lengths, long gestation periods, long periods of caring for young, and an abundance of meat presented to eager hunters. Consequently, even a small increase in mortality may very well result in a large animal’s annual mortality exceeding its generational mortality, the end result being extinction. This relationship also partly explains why present-day elephant populations are unable to withstand poaching in many parts of Africa (Box 7.2).
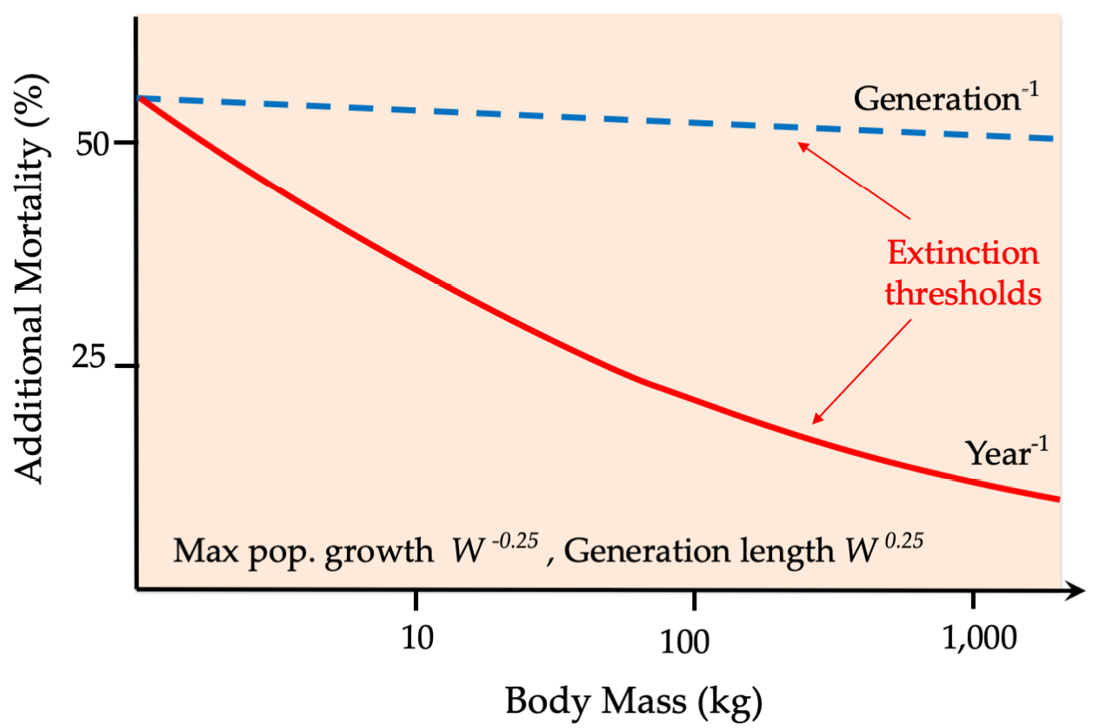
Figure 8.B The impact of additional mortality per year, as it relates to mammalian body mass. The graph shows how even slight increases in annual mortality can rapidly drive megaherbivore species to extinction. After Brook and Bowman, 2005, CC BY 4.0.
The lessons from the Pleistocene extinctions are relevant also today. As explained above, accumulated evidence indicates that early humans have caused extensive ecosystem changes and species extinctions extending over more than a million years. Homo sapiens had emerged in Africa about 300,000 years ago (Callaway, 2017). As early humans mastered the use of fire, poison-tipped spears and arrows, pitfall traps, and a host of additional hunting techniques, this unique apex predator proceeded to influence the structure and composition of African (and global) landscapes, and the plant and vertebrate assemblages of the continent (Smith et al., 2019). For the last two million years our ancestors have set in motion a series of trophic cascades that continue to this day and are resulting in increasing loss of diversity of the flora and fauna of the African continent and the rest of the world.
While early extinctions were generally isolated and selective, extinction rates increased rapidly after the rise of agriculture, and especially after European settlers started colonising Africa from the 17th century onward. By no coincidence, the area where most of the extinctions and extirpations during colonialism occurred was on the southwestern tip of Africa, the location of the earliest intensive European settlements on the continent. For example, by 1700, hunting caused the extirpation of every single land animal over 50 kg within 200 km of Cape Town (Rebelo, 1992). As hunters moved further afield in search of targets, Africa saw its first post-colonial large mammal extinctions, namely the bluebuck (Figure 8.4), quagga (Equus quagga quagga, EX), and Cape warthog (Phacochoerus aethiopicus aethiopicus, EX).
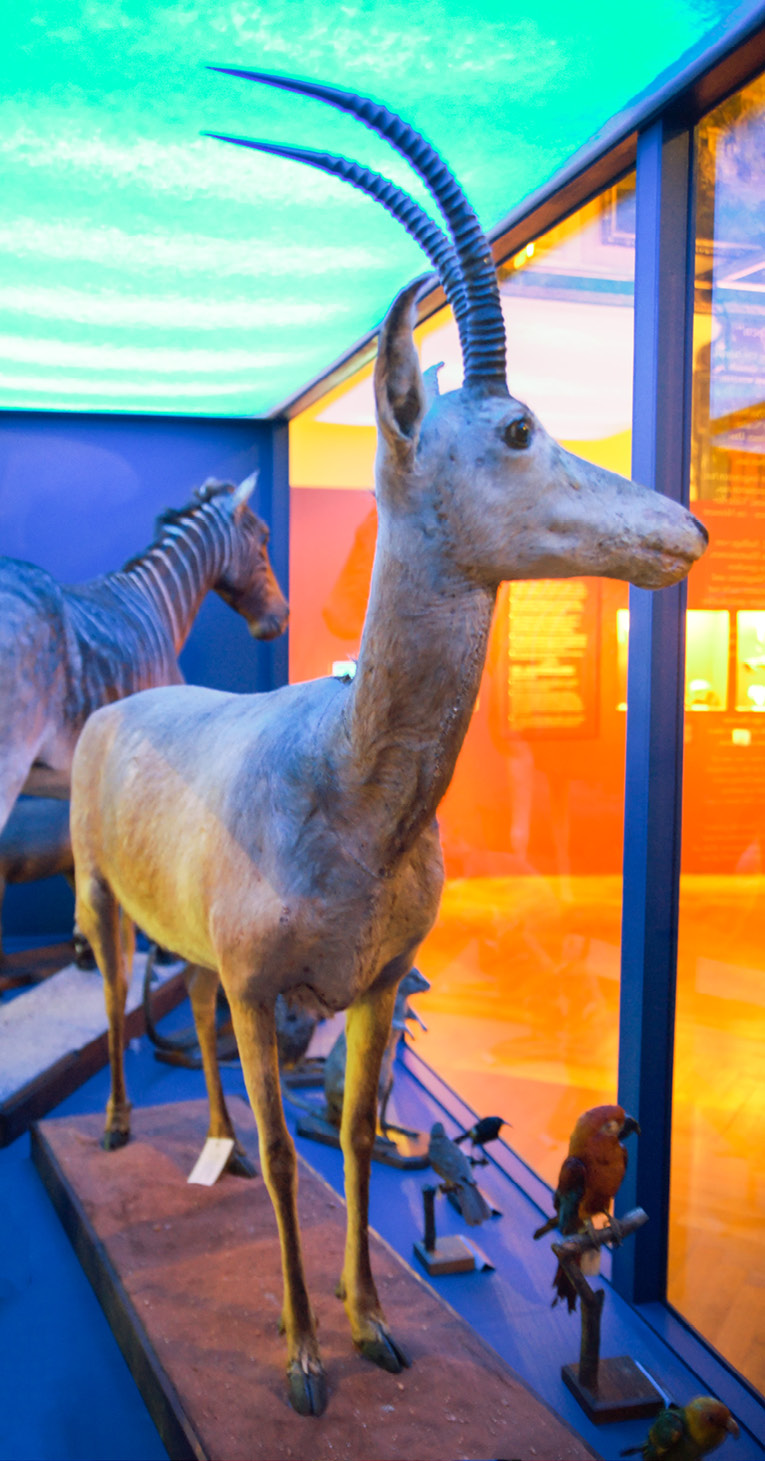
Figure 8.4 One of only four remaining skins of the bluebuck at the Vienna Museum of Natural History, Austria. Once a prized hunting target, it was the first known African antelope hunted to extinction. In the background is a quagga (Equus quagga quagga, EX), another African animal hunted to extinction. Photograph by Sandstein, https://en.wikipedia.org/wiki/Bluebuck#/media/File:Hippotragus_leucophaeus,_Naturhistorisches_Museum_Wien.jpg, CC BY 3.0
Following the demise of many of the Cape Floristic Province’s large animals, humans have driven African species to extinction at an increasing pace. Today, at least 84 Sub-Saharan African species have been confirmed Extinct (Figure 8.5), nine species are Extinct in the Wild, and as many as 202 species are considered possibly Extinct (IUCN, 2019). Among the extinct species are two wildflowers (Acalypha dikuluwensis, EX; Basananthe cupricola, EX) wiped out by mining activities in the DRC; and from Seychelles, an endemic parakeet (Psittacula wardi, EX) that was hunted to extinction. Among the species that persist only in captivity is the Kihansi spray toad (Nectophrynoides asperginis, EW), whose population crashed from more than 20,000 individuals in June 2003 to only five individuals in January 2004 after the establishment of a hydropower plant in eastern Tanzania (Channing et al., 2006). While some species that are Extinct in the Wild may be released back into the wild at some point in future, the four (or possibly seven) cycad species that persist only in captivity will probably not be reintroduced due to ongoing concerns about poaching by plant collectors (Okubamichael et al., 2016).
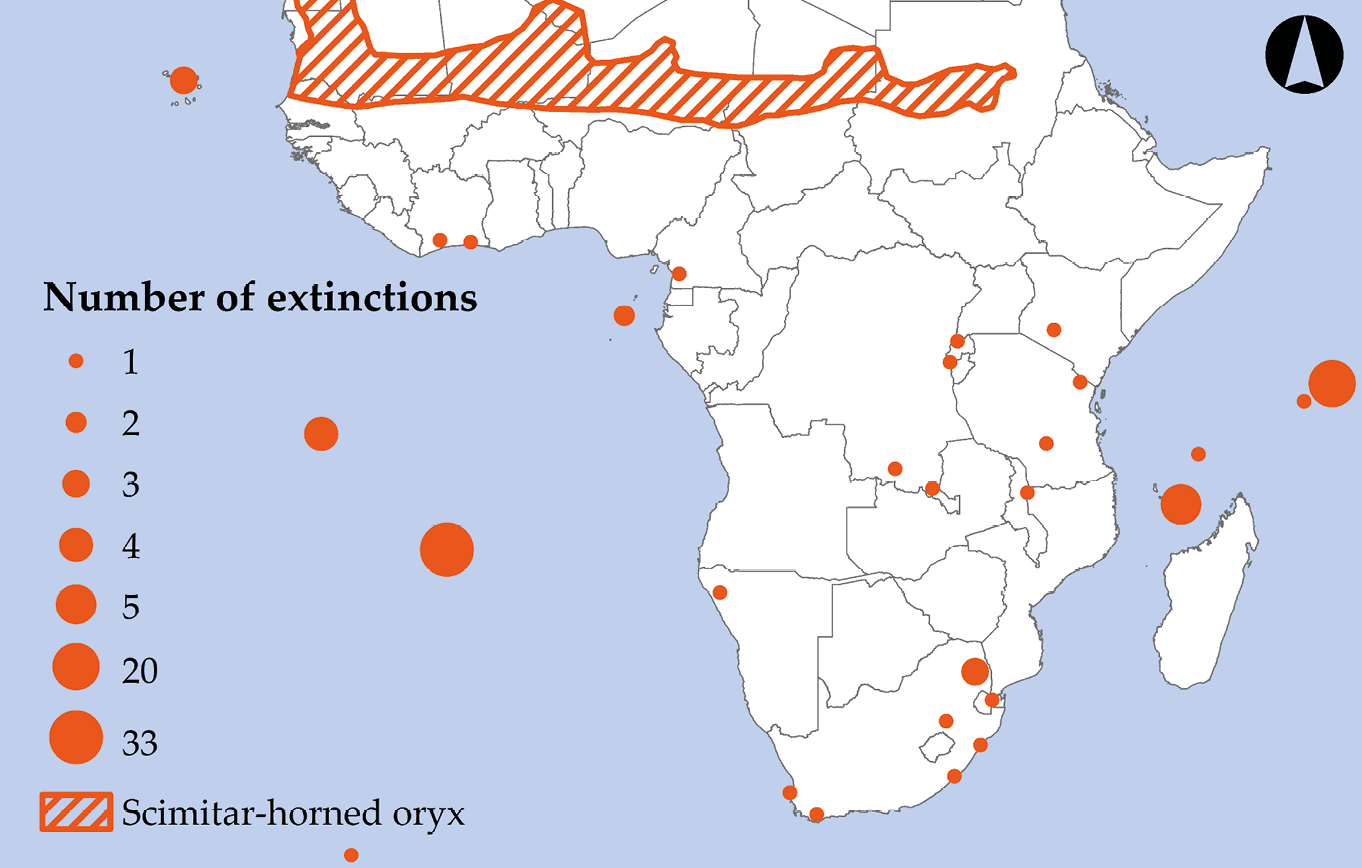
Figure 8.5 The locations of Sub-Saharan Africa’s wildlife extinctions (including Extinct and Extinct in the Wild species) since 1500. Note how the largest number of extinctions involve species with restricted distributions, particularly those that occurred on islands. The scimitar-horned oryx is an example of mainland species that went extinct despite its large original range. Source: IUCN, 2019. Map by Johnny Wilson, CC BY 4.0.
While most of Africa’s extinctions—at least until now—were isolated events involving one or two species at a time, the region also provides one of the best-studied examples of a recent man-made mass-extinction event. In the mid-1950s, the Uganda Game and Fisheries Department introduced the predatory Nile perch (Lates niloticus) to Lake Victoria to bolster the local fishery industry (Pringle, 2005). An ecological and economic disaster followed, pushing the entire ecosystem to the brink of collapse. First, the local people continued to prefer smaller endemic cichlids—which they could preserve by drying in the sun—to the perch with its oily flesh. This allowed the predatory perch’s population to grow unchecked which, in turn, reduced over 500 endemic cichlid species’ populations by 80% in just a few years (Witte et al., 1992). As the cichlid populations crashed, some local people started consuming perch for protein; however, they preferred smoking the perch over wood fires. To obtain firewood and charcoal, trees were logged around the lake, which in turn increased eutrophication, as well as erosion and siltation. Despite this array of emerging ecological threats, the local fishery continued to harvest the rapidly declining cichlid population. Consequently, as many as 200 cichlid species may have been driven to extinction in the decade following the perch introduction (Goldschmidt et al., 1993).
Judging by the number of extirpations over the last few decades, Africa will undoubtedly see more species pushed to extinction in the coming decades. Of particular concern is West and Southern Africa, which have lost over 75% of its large mammal populations over the past few decades; losses across Sub-Saharan Africa as a whole generally amount to over 50% (Ceballos et al., 2017). Some species will hopefully be spared this fate with the help of people and organisations fighting for their continued survival (Box 8.2). Many species will not be so lucky. The world’s last western black rhinoceros (Diceros bicornis longipes, EX) died in Cameroon in 2011; the northern white rhinoceros (Ceratotherium simum cottoni, CR) may follow this fate within the next few years (see Box 11.4). Lions (Panthera leo, EN) have been extirpated from as many as 16 African nations (Bauer et al., 2015), while cheetahs (Figure 8.6) occur in less than 9% of their historic range (Durant et al., 2017).
Box 8.2 Swimming Dangerously Close to Extinction: Population Crash in Lesotho’s Endemic Maloti Minnow
Freshwater Research Centre (FRCSA),
Kommetji, South Africa.
jembejem@gmail.com
Lesotho’s iconic Maloti minnow (Pseudobarbus quathlambae, EN) (Figure 8.C) is a small, stream-dwelling cyprinid, and is the only freshwater fish species endemic to the country. Historically, the species was widespread, but its distribution has become increasingly restricted and fragmented in recent times due to interactions with non-native fishes and habitat degradation (Skelton et al., 2001), leading to it being classified as Endangered by the IUCN (Chakona and Kubheka, 2018). Genetic research has revealed that what was previously considered a single widespread species comprises two genetically distinct lineages: a “Mohale lineage” found in the Mohale catchment, and an “Eastern lineage” which includes populations in five catchments east of the Mohale catchment (Skelton et al., 2001). The genetic divergence between the two Maloti minnow lines is a result of a long period of geographic isolation and warrants that they be conserved as separate evolutionary significant units (ESU). Furthermore, the Mohale lineage, which comprises 77% of the species’ known distribution, is of critical importance for continued survival of the species (Skelton et al., 2001).
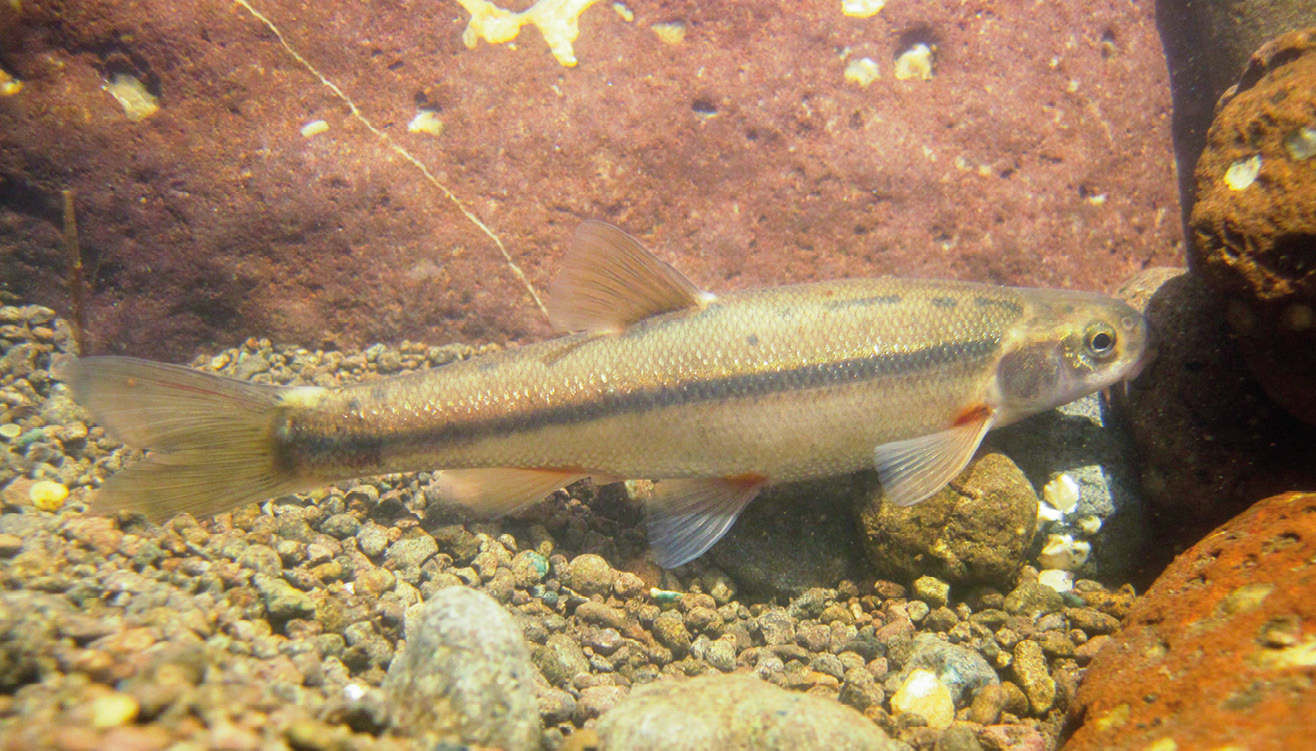
Figure 8.C The Maloti minnow, the only freshwater fish species endemic to the highlands of Lesotho, faces extinction due to habitat degradation and invasive species. Photograph by Craig Garrow, CC BY 4.0.
Past surveys (e.g. Steyn et al., 1996) have revealed that the Maloti minnow was the only fish species inhabiting the rivers flowing into the Mohale Reservoir. Situated 4 km below the Reservoir, the 20 m high Semongkoaneng waterfall has historically prevented larger fish species from moving upstream into the upper catchment. Following the filling of the Mohale Reservoir in 2003, an inter-basin transfer (IBT) tunnel linking it to Katse Reservoir was opened. Biologists working in the catchment subsequently expressed concern that non-native fishes might colonise the Mohale Reservoir via the IBT tunnel, and from there invade the influent rivers (Rall and Sephaka, 2008). Because the Maloti minnow evolved in the absence of large-bodied fishes, it would not have had an opportunity to evolve adaptations to cope with competition from and predation by larger species and may, therefore, be particularly sensitive to the arrival of other fish.
In 2006, the smallmouth yellowfish (Labeobarbus aeneus, LC), a larger, more aggressive cyprinid, was recorded in Mohale Reservoir (Rall and Sephaka, 2008), suggesting that it had dispersed from Katse Reservoir through the IBT tunnel. By 2013, it had spread into the major influent rivers in that system and coinciding with this was a virtual disappearance of the Maloti minnow from this former stronghold for the Mohale lineage. To illustrate this, surveys in previous decades described healthy populations of several thousand fish (e.g. Steyn et al., 1996), while only five individuals were recorded from the same sites in 2013 (Shelton et al., 2017).
Interestingly, the saving grace for this lineage may have originated from the same source that landed them in this predicament in the first place: human intervention. Prompted by the opening of the Kaste-Mohale IBT, a small team of passionate conservation scientists translocated several Maloti minnows to sections of stream above tall waterfalls, upstream of their natural distribution range (Rall and Sephaka, 2008). These sections, they knew, would be unreachable by larger species swimming upstream from Mohale Reservoir. This assisted colonisation approach has been viewed as controversial, but it may also have saved a tiny minnow from almost certain extinction in the wild. The prospect of losing a charismatic species like the Maloti minnow showcases how projects like the Lesotho Highlands Water Project can easily damage sensitive ecosystems that were not consider in development plans. In order to save the Maloti minnow from extinction, the next step will be to assess the success of translocation efforts and develop a rescue plan for the species.
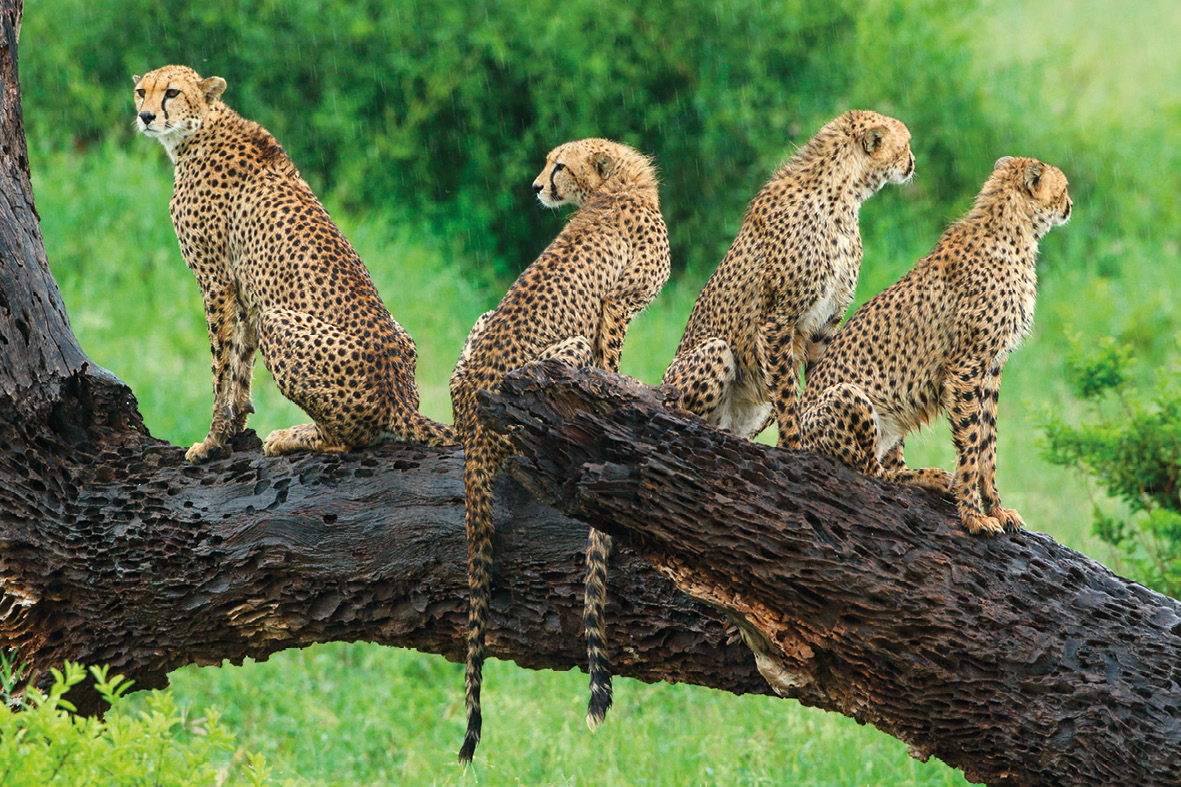
Figure 8.6 A cheetah mother and her cubs in Tanzania’s Tarangire National Park. Once found across much of Africa, cheetahs are now extirpated in 90% of their historic range. Photograph by Markus Lilje, CC BY 4.0.
8.5 Which Species are at Risk of Extinction?
An important task for conservation biologists is to identify and prioritise those species in greatest danger of extinction. Accomplishing this task requires biologists to collect and review all the information we have on each species. To facilitate this major undertaking, the IUCN has formalised the evaluation and reporting of threatened species assessments using an internationally accepted standard of conservation categories to reflect a taxon’s risk of extinction. These nine categories (Figure 8.7), known as Red List Assessments (IUCN, 2017), are:
- Extinct (EX). These species are no longer known to exist. As of mid-2019, the IUCN has listed 84 Sub-Saharan African species as Extinct.
- Extinct in the Wild (EW). These species exist only in cultivation, in captivity, or other human-managed situations. As of mid-2019, the IUCN has listed nine Sub-Saharan African species as Extinct in the Wild.
- Critically Endangered (CR). These species have an extremely high risk of going extinct in the wild. As of mid-2019, the IUCN has listed 880 Sub-Saharan African species as Critically Endangered. Also included in this category are the 202 Sub-Saharan African species that the IUCN considered possibly Extinct.
- Endangered (EN). These species have a very high risk of extinction in the wild. As of mid-2019, the IUCN has listed 1,600 Sub-Saharan African species as Endangered.
- Vulnerable (VU). These species have a moderately high risk of extinction in the wild. As of mid-2019, the IUCN has listed 2,153 Sub-Saharan African species as Vulnerable.
- Near Threatened (NT). These species are close to qualifying for a threatened category but are not currently considered threatened. As of mid-2019, the IUCN has listed 1,034 Sub-Saharan African species as Near Threatened.
- Data Deficient (DD). Inadequate information exists to determine the risk of extinction for these species. As of mid-2019, the IUCN has listed 2,441 Sub-Saharan African species as Data Deficient.
- Least Concern (LC). These species are not considered Near Threatened or threatened. (Widespread and abundant species are included in this category.) As of mid-2019, the IUCN has listed 11,776 Sub-Saharan African species as Least Concern.
- Not Evaluated (NE). Species that have not yet been evaluated. Most species fall in this category.
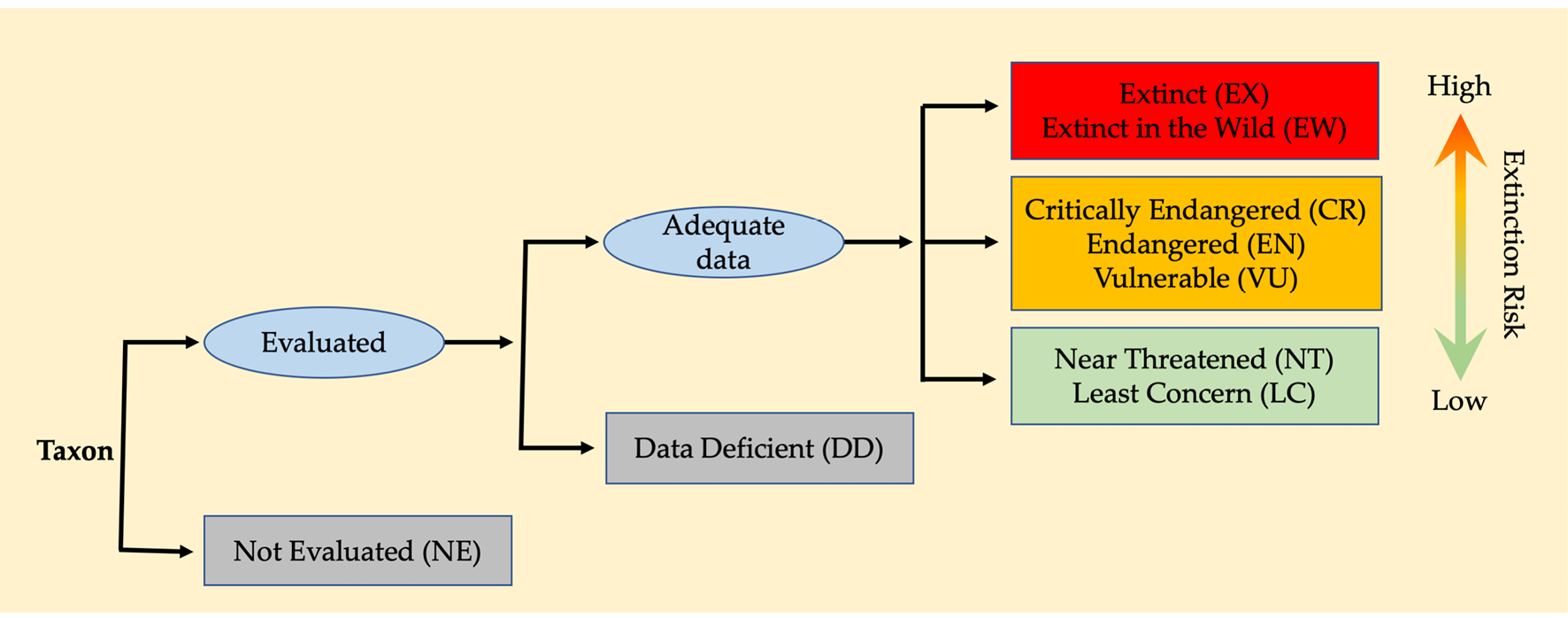
Figure 8.7 Flow diagram illustrating the structure of the IUCN categories of conservation status. An evaluated species can be considered at lower risk of extinction, at high risk of extinction (i.e. threatened), or extinct. A species for which not enough data are available for evaluation is considered Data Deficient (DD). After IUCN, 2017, CC BY 4.0.
These categories, and the Red List Criteria (Table 8.1) used to classify each species, are broadly based on population viability analysis (Section 9.2), and consider population size, population trends, and habitat availability. Species that are Extinct in the Wild, Critically Endangered, Endangered, and Vulnerable categories are officially considered “threatened with extinction”. The advantage of this system is that it provides a standard protocol by which decisions can be reviewed and evaluated according to widely accepted yet flexible criteria. Consequently, species, subspecies, varieties, populations, and subpopulations can be assessed on a global or regional level, all under a unified set of standards. The resultant threat status assessment forms the basis of Red Data Books and Red Lists: detailed lists of threatened wildlife by group and/or by region compiled by the IUCN and its affiliate organisations. All global (and many regional) Red List assessments are freely available at http://www.iucnredlist.org, with feedback links provided from which anyone can alert the IUCN if they find errors or have suggestions for improvements.
Table 8.1 The IUCN’s Red List criteria for evaluating a taxon’s threat status. A species that meet any one of criteria A–E could be classified as Critically Endangered.
Red List criteria A–E |
Summary criteria used to evaluate a taxon as Critically Endangereda |
A. Population size declining |
The population size has declined by 90% (or more) over last 10 years or 3 generations (whichever is longer). |
B. Geographical range declining |
The species is restricted to < 100 km2 and it occurs at a single location and its distribution range is observed/expected to decline. |
C. Small and declining populations |
There are less than 250 mature individuals left and population has declined by 25% (or more) over last 3 years or 1 generation (whichever is longer). |
D. Small populations |
There are less than 50 mature individuals left. |
E. Population viability analysis |
There is a 50% (or greater) chance of extinction within 10 years or 3 generations. |
a Additional criteria for Critically Endangered, as well as criteria for Endangered and Vulnerable listings can be found at http://www.iucnredlist.org.
While nearly 20,000 Sub-Saharan African species have been evaluated as of mid-2019 (IUCN, 2019), these assessments only cover a small proportion of the region’s overall biodiversity. Consider for example that as of mid-2019, just over 4,900 Sub-Saharan Africa’s plants have been listed on the IUCN Red List website. Yet, the Cape Floristic Region alone hosts over 6,200 endemic plant species. The assessment gaps are even more conspicuous for lesser-known taxa; for example, only seven species of bryophytes (a group of non-vascular plants that includes mosses) have been assessed as of mid-2019; some readers of this textbook will have more bryophyte species in their gardens. The reasons for such assessment gaps are many, but most boil down to manpower and funding limitations, which restrict our ability to obtain the data needed for comprehensive assessments. It is thus important to understand that the lack of information on these and other poorly known groups does not mean there is no threat. For example, as of mid-2019, no African abalone (Haliotis spp.) have been assessed, even though these highly valued molluscs are some of Africa’s most heavily exploited (and heavily poached) marine organisms (Minnaar et al., 2018). A lack of information about a species’ threats and populations trends is thus a good argument that more studies are needed, sometimes urgently. Similarly, continued monitoring of species thought to be common is also important, as it can shed light on how new threats may emerge or escalate over time.
8.5.1 Course-filter assessments
To fill Red List species assessment gaps, conservation biologists are increasingly relying on broader metrics, or coarse-filter assessments, to identify groups of species that are threatened with extinction. One such method, which reduces the need to evaluate every individual species, is to identify ecosystems that are threatened. This premise rests on the assumption that any threatened ecosystem will contain many threatened species. Hence, protecting and restoring threatened ecosystems will simultaneously allow many populations living in those ecosystems to recover. To facilitate this type of coarse-filter assessment, the IUCN recently established a Red List of Ecosystems (RLE, http://iucnrle.org). The RLE assesses ecosystem status against five criteria: (1) distribution declines, (2) distribution restrictions, (3) environmental degradation, (4) disruption of ecological processes and interactions, and (5) quantitative estimates for risk of ecosystem collapse (Keith et al., 2013). While the ecosystem assessment protocol was only recently developed—only three African ecosystems have been assessed as of mid-2019—its holistic strategy promises a more comprehensive accounting of local biodiversity which could be more informative than an accumulation of single species assessments.
8.6 Characteristics of Threatened Species
While a great number of factors may make a species vulnerable to extinction, conservation biologists have observed that species most vulnerable to extinction generally fall under one of six main groups:
- Species with small populations: Some species have very small populations, consisting of just a few individuals. Such small populations are highly vulnerable to random variations in demography or environmental conditions, and to the loss of genetic diversity—all factors that increase the risk of extinction (Section 8.7). Species whose population sizes naturally fluctuate between large and small populations also fall in this category, as they are at an increased risk of extinction during the small population phases of those fluctuations.
- Species with declining populations: Trends in population sizes tend to persist, so populations that are declining in abundance face a high risk of extinction (Caughley, 1994) unless conservation managers identify and address the causes of decline. Species impacted by the threats discussed in Chapters 5–7 generally also have declining populations.
- Species with restricted distribution ranges: Some species, such as those that are restricted to oceanic islands; mountains peaks; or isolated lakes, can be found only in a limited geographic range. A major disturbance, such as a cyclone/hurricane or drought, could easily affect that entire species’ range, potentially driving the species to extinction.
- Species with only one or a few populations: A sufficiently large disturbance—such as a wildfire, storm, or disease outbreak—can wipe out a single population of a species. For a species with only one population, that means its extinction, while the loss of even a single population leaves species with only a few populations more vulnerable to the next disturbance. Species in this category (few populations) overlap with those in the previous category (restricted distribution ranges) because species with few populations tend to have restricted ranges.
- Species that are exploited by people: Overharvesting can easily reduce a population to the point of extinction (Section 7.2). Even if overharvesting is stopped just before the point of extinction, it may still have reduced a population to a size where it becomes susceptible to one or more of the three additional pressures faced by small populations (Section 8.7).
- Species with critical symbiotic relationships: Species that are members of obligate symbiotic relationships (where one species cannot survive without another) will go extinct if its host disappears. For instance, larvae of the rhinoceros stomach botfly (Gyrostigma rhinocerontis) mature in the stomach lining of African rhinoceros, and no other species (Barraclough, 2006). Thus, if the host species (the rhinoceros) were to go extinct, so would the botfly, Africa’s largest fly species. This phenomenon in which one species’ extinction leads to the extinction of other is called a coextinction (Koh et al., 2004), while a series of linked coextinctions is called an extinction cascade (Section 4.2.1).
The following characteristics are also linked with extinction, although the links are not as strong as is the case with the previous six categories:
- Animal species with large body sizes: Large animals generally require large ranges and more food, have lower rates of reproduction, and have smaller population sizes relative to smaller animals. Often, they are harvested by humans for material benefits (see Box 8.1). Consequently, within groups of related species, the largest are generally also the most vulnerable to extinction—that is, a larger species of carnivore, ungulate, or whale is more likely to go extinct than a smaller carnivore, ungulate, or whale.
- Species that require a large home range: Individuals or social groups of some species must forage over wide areas to fulfil their needs. When portions of their range are being degraded or fragmented, the remaining area will eventually be too small to support a viable population.
- Species that are poor dispersers: Moving to more suitable habitat is a common survival response following altered environmental conditions. But species with poor dispersal abilities may be doomed to extinction if they are unable to move to more suitable areas elsewhere (see e.g. discussion on range-shift gaps, Section 6.3.5).
- Seasonal migrants: A migratory species depends on intact ecosystems at two or more locations to complete its life cycle (see Box 5.3). If those ecosystems, either at stop-over sites along migration routes and/or at migratory endpoints, are damaged, the species may be at risk of extinction.
- Species with low genetic diversity: Because genetic diversity (Section 3.2) enables species to adapt to changing environmental conditions, species with low genetic diversity are more vulnerable to extinction because they have less ability to adapt to new diseases, new predators, or recent changes in their ecosystems.
- Species that evolved in stable ecosystems: Species that evolved in relatively stable environments (e.g. tropical ecosystems) are often threatened with extinction because under stabile conditions, a species is unlikely to retain the ability to adapt to environmental changes such as altered microclimates.
- Species with specialised requirements: Specialist species are often threatened with extinction because they are unable to adapt to altered ecosystems.
- Group-living species: A range of factors leaves group-living species at risk of extinction. For example, a herd of ungulates, a flock of birds at their night-time roost, or a school of fish can be harvested in its entirely by people using highly effective techniques. Even if some individuals remain, the harvesting may still leave the population below a critical threshold needed for effective foraging, mating, or territorial defence. This link between population size/density and individual fitness is termed the Allee effect (Section 8.7.2).
- Species that have had no prior contact with people: Species that encounter people for the first time are ecologically naïve—they lack avoidance strategies that promote survival during these encounters. Ecologically naïve species thus have a higher chance of extinction than species that have already survived human contact.
- Species closely related to species that recently went extinct: Groups of closely-related taxa, where some members are threatened or already extinct, often share characteristics that elevate their threat of extinction. Groups of related taxa that include many threatened species include apes, cranes, sea turtles, and cycads.
- Species that live on islands: Island species generally exhibit many of the characteristics mentioned above. In addition, the mere fact that an island is surrounded by ocean means that species that are unable to swim or fly have nowhere to go when they need to escape a threat.
8.7 Problems of Small Populations
While some small populations have persisted against the odds, sufficiently large populations are generally needed to prevent eventual extinction (Halley et al., 2016, see also Section 9.2). Small populations—which include species that have always had small populations and previously large populations that have been reduced to a few individuals—face three additional inherent and unavoidable pressures beyond the threats discussed in Chapters 5–7. These three additional pressures are: (1) loss of genetic diversity; (2) demographic stochasticity; and (3) environmental stochasticity and natural catastrophes. We will now examine how each of these pressures can lead a small population to eventual extinction. Much of this discussion is based on a ground-breaking manuscript by New Zealand ecologist Graeme Caughley, which discusses at length the threats faced by small and declining wildlife populations (Caughley, 1994).
8.7.1 Loss of genetic diversity
Species with high genetic diversity are generally more able to adapt to and reproduce under new conditions such as those brought by environmental changes (Section 3.2). These adaptations can occur at both individual and population levels. For example, under climate change, some genes may allow some populations to adapt their ranges faster or better tolerate warmer and wetter environments, while phenotypic plasticity—the ability of one gene to express itself differently under different conditions—may allow certain individuals to better adapt to a changing environment. One species that displays remarkable phenotypic plasticity is the crystalline iceplant (Mesembryanthemum crystallinum); by regulating its photosynthetic pathways, an individual plant can adjust its water needs based on the amount of salt and moisture available in the environment (Tallman et al., 1997). Such flexibility may explain why this species, native to southwestern Africa, North Africa, and Europe, has been a successful invader in environments as diverse as those in South America, North America, and Australia.
While populations with many individuals usually also have high levels of genetic diversity, small populations regularly suffer from low levels of genetic diversity. This low genetic diversity not only leaves those populations unable to adapt to changing conditions, but also makes them more susceptible to a variety of deleterious genetic effects (Caughley, 1994). Each of these effects leads to even greater loss of fitness and genetic diversity, hence even larger population declines, and eventually extinction. In the next sections, we discuss further why these deleterious genetic effects are so harmful to small populations.
Genetic drift
In wildlife populations, there are always some alleles that are relatively common, and others that are relatively rare. The relative abundance of any of these alleles may however change from one generation to another purely by chance. While common alleles generally tend to stay common, rare alleles have a high chance of being randomly lost in subsequent generations. Consider how each parent only passes on half of their genetic code to each offspring; this means that the ability of a rare allele to persist is dependent on how many individuals carry it, which individuals produce offspring, and how many offspring those individuals produce. Another important factor is population size (Figure 8.8): in any small population, only a limited number of individuals can carry any single allele, so the smaller the population, the higher the likelihood that alleles are lost to the next generation. This loss of alleles is called genetic drift.
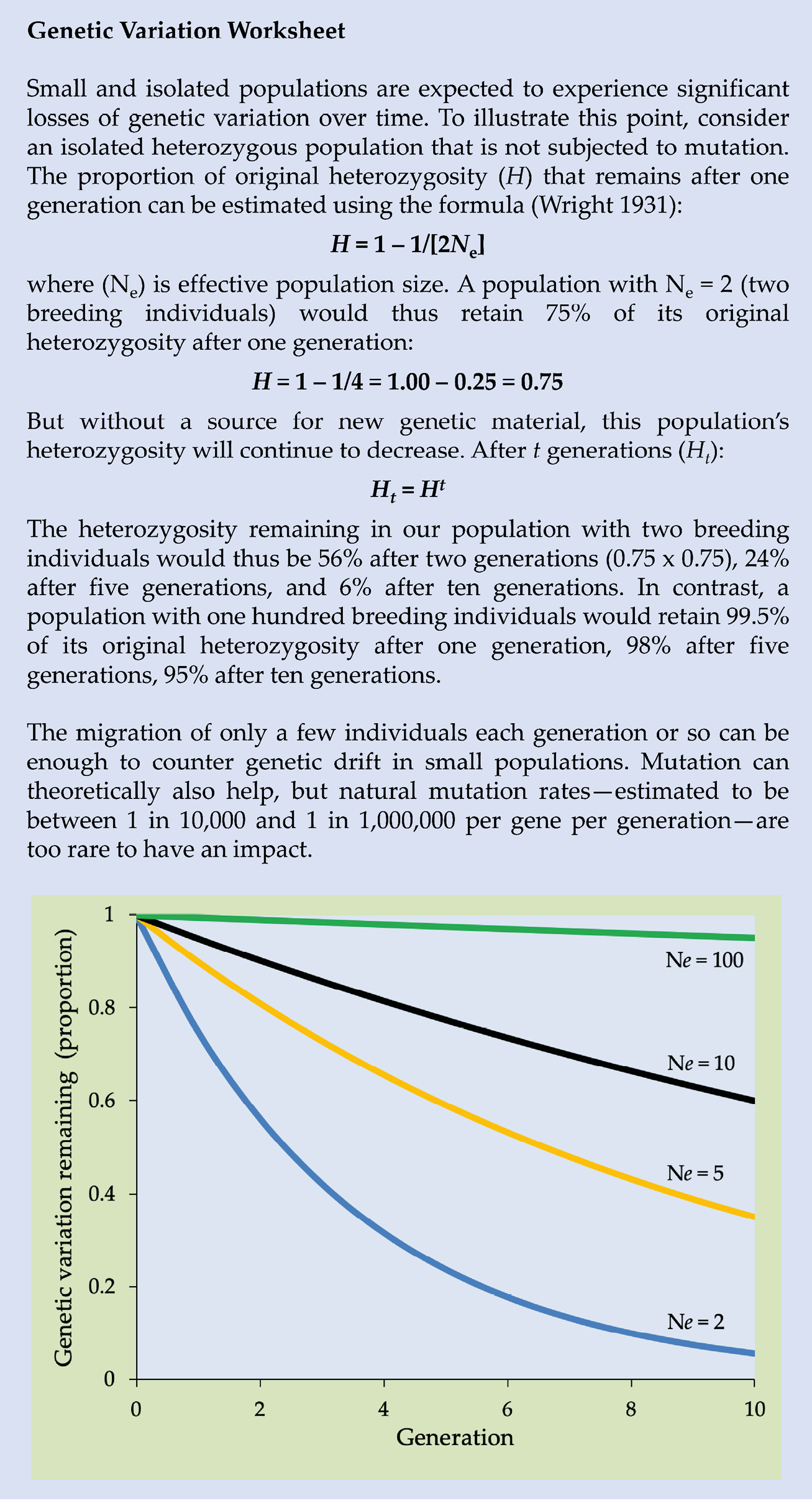
Figure 8.8 The amount of genetic diversity that is randomly lost over time due to genetic drift is highly dependent on a population’s effective population size (Ne). A theoretical population with Ne = 2 may lose approximately 95% of its genetic diversity over 10 generations, while a population with Ne = 100 may lose only 5%. After Meffe and Carroll, 1997, CC BY 4.0.
While genetic drift equates to a loss of genetic diversity, there are some cases where populations show no obvious ill effects. Such may have been the case for female elephants in South Africa’s Addo Elephant National Park. Hunting once nearly killed off this entire population; by the time they were adequately protected in 1931, only 11 animals remained, eight of which were female. Of those eight females, at least four were tuskless, while only two, maybe three, females carried both tusks. Over the next decades, Addo’s female elephants have shown increasing degrees of tusklessness; by 2002, only 2% of females had tusks (by comparison, 96–98% of elephant females are normally expected to develop tusks, Maron, 2018). One can therefore postulate that the allele responsible for the tusk development in female elephants became rare, and that the progressive loss of tusked females is a sign of genetic drift (Whitehouse, 2002). While Addo’s female elephants do not show any known limitations from being tuskless, the loss of alleles can also be devastating to the population suffering from genetic drift if, for example, the lost allele(s) coded for traits that would have allowed a species to adapt to a changing environmental condition.
It is important to note that genetic drift is distinct from natural selection. That is, genetic drift involves random changes in the frequency of alleles, whereas natural selection involves changes in traits in response to sexual selection or specific environmental conditions. For example, reduced tusk size in some heavily-hunted elephants in Africa (e.g. Chiyo et al., 2015) is a selective pressure in response to hunting that favour large tusks—this is distinct from Addo’s female elephants that have lost their tusks even in the absence of selective hunting pressure.
Mating among closely related individuals, which occurs in small populations, often results in lower reproductive success and weaker offspring.
Inbreeding depression
In large populations, a variety of instinctive mechanisms are in place to promote heterosis, which occur when offspring have a level of genetic variation that improves their individual evolutionary fitness. Some species are predisposed to disperse from their place of birth to prevent sibling–sibling or parent–offspring mating, while others are restrained from mating with close relatives through sensory cues such as individual odours. Many plants have morphological and physiological traits that facilitate cross-pollination and reduce self-pollination.
However, in small populations with few unrelated mates, the urge to breed might be stronger than the mechanisms that promote heterosis. Under these conditions, rather than forgoing reproduction, breeding among closely-related individuals (or inbreeding) can occur. This breeding among close relatives might result in inbreeding depression, which can occur when closely-related parents give their offspring two copies of a deleterious allele. Individuals suffering from inbreeding depression typically have fewer offspring or have offspring that are weak or fail to reproduce. Such is the case for some mountain gorillas (Gorilla beringei beringei, EN): genetic studies have shown how birth defects in several small populations can be attributed to inbreeding depression (Xue et al., 2015). Inbreeding depression has also been identified as the reason why some small lion populations are more susceptible to diseases (Trinkel et al., 2011). Inbreeding depression can result in a vicious cycle for declining population sizes, where such declines can lead to even more inbreeding depression, and eventually extinction (see Section 8.7.4).
Outbreeding depression
Large populations have many ecological, behavioural, and physiological mechanisms that prevent hybridisation, the production of offspring among genetically distant taxa, whether they be individuals of different species, or individuals of the same species but with different adaptations (the latter being intraspecific hybridisation). As with inbreeding depression, these mechanisms may fail in small populations, leading to outbreeding depression (Frankham et al., 2011). Because offspring that result from outbreeding depression have traits that are intermediate to their parents, they may not be adapted to either of the parents’ ecosystems. For example, one study found that plants suffering from outbreeding depression have weakened defences against herbivory (Leimu and Fischer, 2010). Outbreeding depression may also lead to a breakdown in physiological and biochemical compatibility between would-be parents—hybrid sterility is a well-known consequence of this breakdown. Consequently, species and populations suffering from outbreeding depression often show similar symptoms to inbreeding depression, including lower fitness, weakness, and high rates of mortality.
The opposite of outbreeding depression is hybrid vigour. Under these conditions, the hybrid offspring can be quite strong in an evolutionary sense; they may even outcompete their parent species. Such is the case with the South African endemic black wildebeest (Connochaetes gnou, LC); having recovered from near-extinction, poorly planned translocations are now threatening this species, which readily hybridises with the widespread common wildebeest (Connochaetes taurinus, LC) in areas of contact (Grobler et al., 2011).
Population bottlenecks
In some taxa, such as butterflies, annual plants, and amphibians, population size varies dramatically from generation to generation. During some years, populations can be so large that they appear to face little risk of extinction. However, abundant years can be misleading when followed by successive years of low abundance. Generally, in a population that undergoes extreme size fluctuations, the population size required to ensure continued persistence (i.e., the minimum viable population (MVP), Section 9.2) is in effect much nearer the lowest than the highest number of individuals in any given year. However, during years with low abundance, a phenomenon known as a population bottleneck may occur—that is, the small population size may lead to the loss of rare alleles from one generation to the next. Population bottlenecks may lead to more inbreeding depression which, in turn, reduces reproductive success (Heber and Briskie, 2010) and increases vulnerability to diseases (Dalton et al., 2016). Low genetic diversity in great white sharks (Carcharodon carcharias, VU) living in South Africa’s Indian Ocean is thought to be the result of a population bottleneck (Andreotti et al., 2015).
Populations founded by only a few individuals by definition start off with low genetic diversity, having lasting effects in the population through time.
New populations founded by only a few individuals are vulnerable to a special type of population bottleneck, the founder effect. The founding individuals of a new population by definition start off with low genetic diversity, much less than the original population that the founders left behind. This low genetic diversity puts the new population at risk of further genetic diversity declines, which have lasting effects through time. This situation can occur naturally when only a small number of individuals disperse to establish a new population or when founder individuals come from a small population that already suffered from low genetic diversity. Being mindful of these concerns is especially important for translocation (Section 11.2) or captive breeding (Section 11.5) projects. For example, to prevent extinction of the world’s smallest gazelle, the Speke’s gazelle (Gazella spekei, EN), a captive population of this species, almost entirely restricted to Somalia, was established in the USA. The founder population for this captive breeding project consisted of only one male and three females, leading to severe levels of inbreeding depression and high mortality rates in offspring (Kalinowski et al., 2000). Understanding the importance of managing for genetic diversity can help avoid these and other challenges that can threaten the success of translocation projects.
8.7.2 Demographic stochasticity
Demographic stochasticity (also known as demographic variation) refers to random variations in a population’s demographic traits (e.g. sex ratios, birth rates, death rates), the cumulative effect of variation in individual organisms’ fitness. In any natural population, some individuals will produce fewer offspring than average, while others will produce more than average; some individuals will produce no offspring at all. Similarly, some individuals die younger than average, while others live longer than average. For populations that are sufficiently large, average birth and death rates provide relatively stable descriptions of key aspects of that population’s demography. However, when a population’s size decreases to below a certain threshold, variations in fitness of a small number of individuals can have a large impact on the overall populations’ demographic parameters, causing population size and other characters to fluctuate up or down unpredictably (Schleuning and Matthies, 2009). Consider, for example, an isolated population of crocodiles with only a few females. As with many other reptiles, offspring sex ratios of crocodiles are determined by the environmental temperature during incubation (Hutton 1987). If, by chance, the population experiences two years of high temperatures, which favour male offspring, and the few females die by chance, the all-male population may be doomed for extinction unless some female crocodiles immigrate from elsewhere.
The social systems of group-living animals can easily be disrupted when their population size or density falls below a critical level.
Small population sizes or low densities can also disrupt social interactions among individuals—especially interactions that affect reproduction—which can cause populations to become demographically unstable. This situation, referred to as the Allee effect, can result in further declines in population size, population density, and population growth rate. Obligate cooperative breeders, such as African wild dogs (Lycaon pictus, EN), are especially vulnerable to the Allee effect (Courchamp et al., 2000) since they need a certain number of individuals to protect their territories and obtain enough food for their offspring (Figure 8.9). Allee effects might also prevent impact group-living species that are not cooperative breeders—recalling the “safety in numbers” mantra, Allee effects seem to prevent the recovery of locally-rare sable antelope (Hippotragus niger, LC) populations in South Africa’s Kruger National Park, as reduced herd sizes increases their exposure to predation (Owen-Smith et al., 2012). But even solitary species that live at low densities are susceptible to Allee effects, since they may find it hard to locate mates once the population density drops below a certain level.
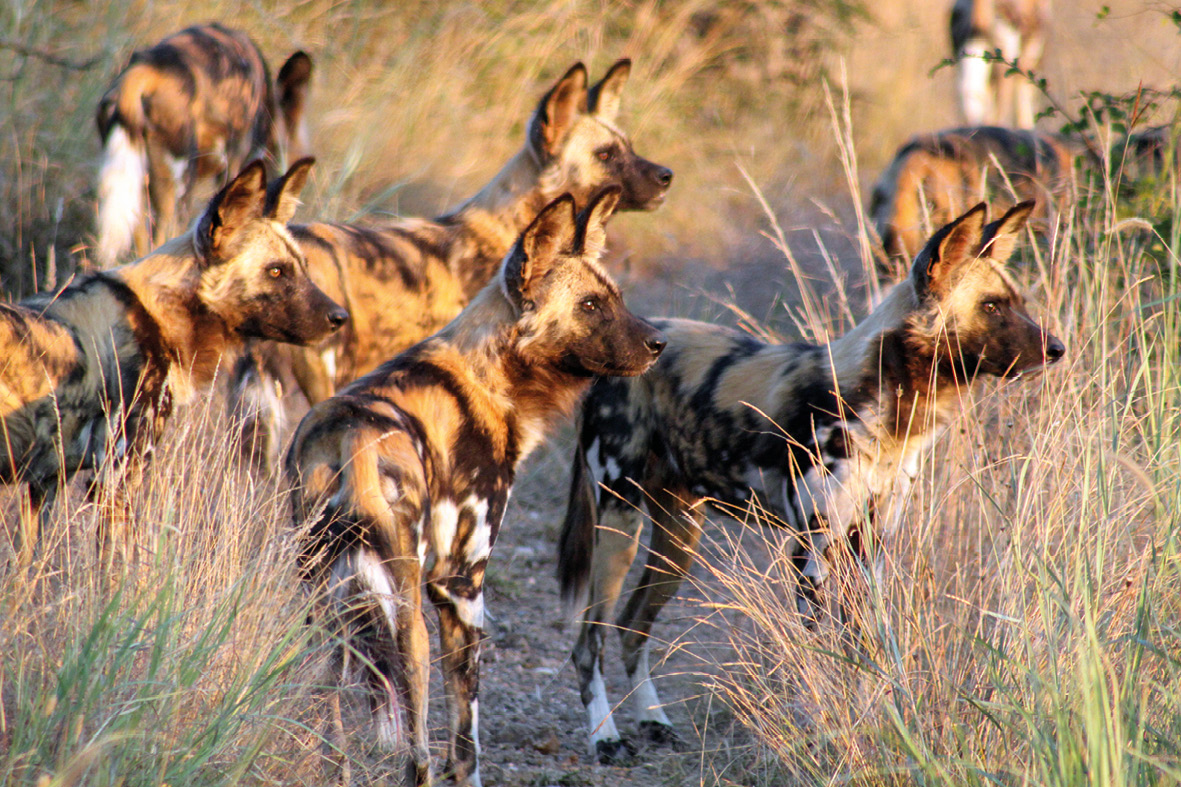
Figure 8.9 A pack of African wild dogs on a hunt in Madikwe Game Reserve, South Africa. Due to their vulnerability to Allee effects, African wild dog populations can only be sustained if packs are above a certain threshold that allows them to hunt, feed their young, and protect themselves effectively. Photograph by flowcomm, https://www.flickr.com/photos/flowcomm/13945572529, CC BY 2.0.
8.7.3 Environmental stochasticity and catastrophes
Environmental stochasticity, the unpredictable variation in environmental conditions, can cause dramatic population size fluctuations over time, and hence, substantially increase the risk of extinction. Consider, for example, how the development rate of many insects is strongly temperature-dependent (e.g. Rebaudo and Rabhi, 2018). In an average or warm year, young insects that hatch on time and feed well may result in ecologically fit adults that produce many young, whereas unusually cold years might reduce hatching success and larval activity, which could also reduce adult fitness (Gibert et al., 2001). So, highly unfavourable conditions in any one year can cause dramatic population declines, or even push a species to extinction if conditions persist over successive years across its range.
Even though a small population may appear to be stable or increasing, an environmental catastrophe can severely reduce population size or even cause extirpation or extinction.
The increased risk of extinction from environmental stochasticity also applies to natural catastrophes that can occur at unpredictable intervals (e.g. droughts, storms, earthquakes, and fires). Range-restricted species are particularly vulnerable to this kind of threat. For example, the biodiversity living in and around several African crater lakes are vulnerable to a rather unique natural phenomenon called “lake burping”. Volcanic chambers underneath some of these lakes are rich in CO2. Small amounts of CO2 may sometimes (or constantly, in some cases) seep up through the lake bed into the surrounding water. Because these lakes are thermally stratified—layers of cold, dense water settle near the bottom while warm, less dense water floats near the top—the CO2-saturated water remains near the bottom of the lake. However, when there is a geologic disturbance, such as a landslide or earthquake, massive amounts of CO2 may suddenly be released, first saturating the warmer water at higher levels with CO2 (killing fish and other oxygen-dependent species in the process), before displacing the breathable surface air in and around the lake. In 1986, one such CO2 eruption killed 1,800 people and 3,500 heads of livestock near Cameroon’s Lake Nyos (Krajick, 2003). Some scientists fear that increased deforestation (which may trigger erosion and landslides) and hydraulic fracturing (which may trigger earthquakes, Section 7.1.1) could trigger similar events at other crater lakes in the region.
Environmental stochasticity tends to increase the probability of extinction more than does demographic stochasticity. As discussed, this is especially true for small populations and range-restricted species.
8.7.4 The extinction vortex
As populations decline in size, they become increasingly vulnerable to the combined impacts from the loss of genetic diversity, inbreeding depression, Allee effects, environmental stochasticity, and demographic stochasticity. All these factors tend to lower reproduction, increase mortality rates, and reduce population size even more, in turn driving populations to extinction at increasingly faster rates over time (Fagan and Holmes, 2006). Conservationists sometimes compare this phenomenon to a vortex, spiralling inward, moving faster (or declining faster in the case of a population) as it gets closer to the centre. At the centre of this extinction vortex (Gilpin and Soulé, 1986) is oblivion—the extinction of the species (Figure 8.10).
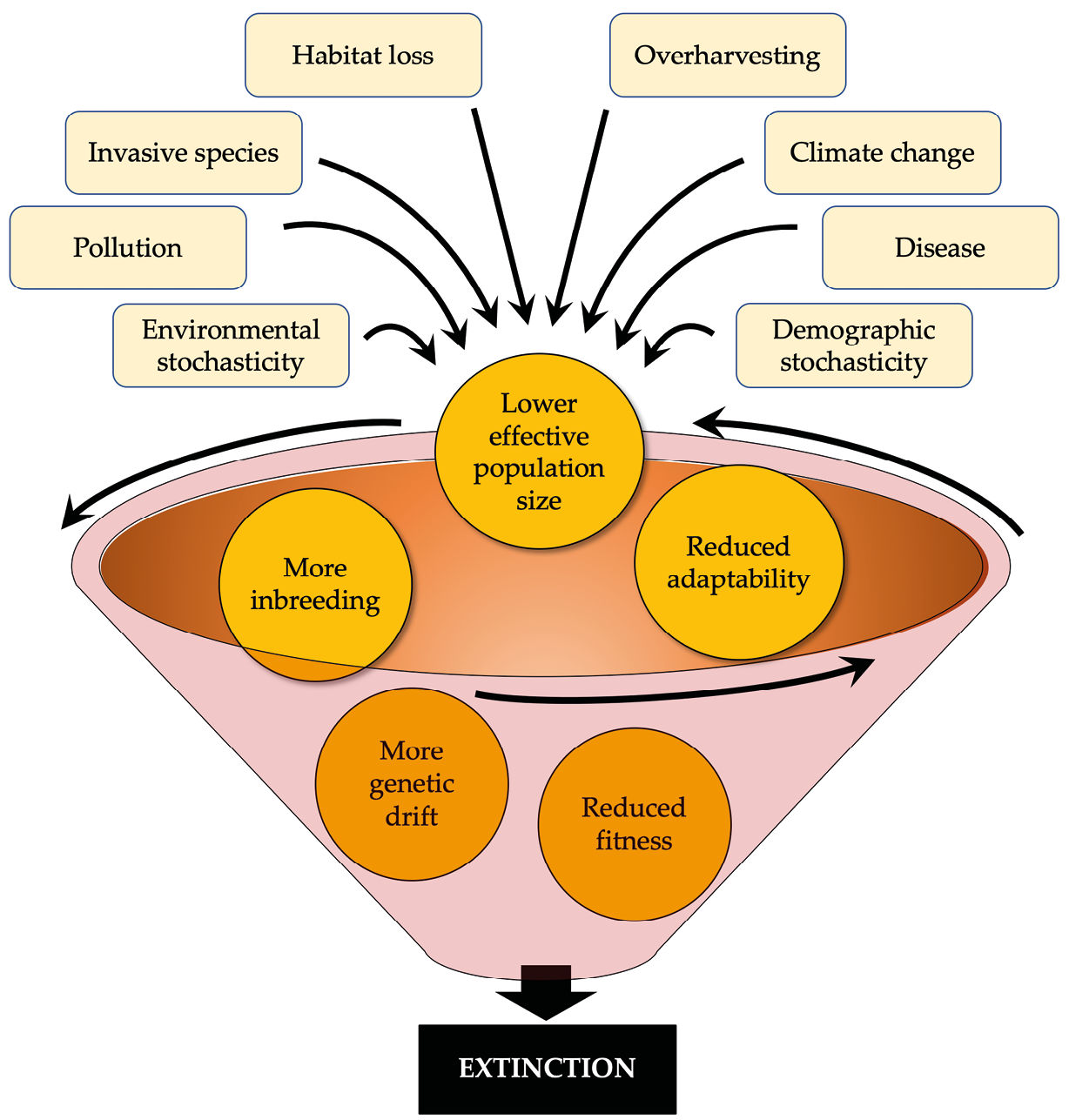
Figure 8.10 The extinction vortex describes a process whereby the factors that affect small populations can drive its size progressively downward towards extinction. CC BY 4.0.
The demise of the bluebuck—the first large mammal of Africa to face this fate after European colonisation—may have been the result of an extinction vortex. When European colonists first arrived in South Africa, this ungulate already persisted as a single, small population of an estimated 370 individuals (effective population size at 100 individuals) and a highly restricted (4,300km2) distribution. Considering this small and restricted population’s vulnerable to deleterious genetic factors and demographic stochasticity, a recent study showed that this species was probably caught in an extinction vortex by the time the first colonist shot the first bluebuck (Kerley et al., 2009). This species would thus likely have gone extinct even in the absence of hunting and habitat loss, which only hastened its departure.
8.7.5 Is there any hope for small populations?
Despite the odds and the many threats facing Africa’s wildlife, many species that were once on the brink of extinction have clawed their way back from the abyss towards stable, and sometimes even growing populations. Prime examples include the Pemba flying fox (Pteropus voeltzkowi, VU); considered Critically Endangered in 1996, conservation education programmes raised awareness of this unique bat, which now has considered Vulnerable, having recovered to more than 28,000 individuals (Entwistle and Juma, 2016). Similarly, because of habitat destruction and introduced predators, the future of the Seychelles magpie-robin (Copsychus sechellarum, EN) looked rather bleak in 1970, when only 16 individuals remained, all on one island. Today, thanks to habitat restoration efforts, supplemental feeding, invasive species eradication, provisioning of nest boxes, and a translocation programme, there are more than 280 Seychelles magpie-robins scattered across five islands (Burt et al., 2016). Another remarkable conservation success story involves the rescue of the southern white rhinoceros (Ceratotherium simum simum, NT), which was reduced to about 20 individuals in a single protected area in the late 1880s. Dedicated conservation efforts since then have seen this iconic species recover to more than 20,000 individuals, with individuals introduced and reintroduced all over Africa and zoos throughout the world. None of these species would have been alive today if it wasn’t for intensive multi-year efforts by dedicated conservation biologists to pull them out of their individual extinction vortices.
Bringing species with small populations back from the edge of extinction requires dedication, careful planning, and significant amounts of resources.
Bringing species with small populations back from the edge of extinction requires dedication, careful planning, and significant amounts of resources. It also requires careful population management to mitigate the negative impacts of founder effects and both demographic and environmental stochasticity (Box 8.4; see also Chapter 11). As these examples show, it can be done. But, given the challenges, it should always be a priority to prevent a species from declining to very low numbers in the first place.
Box 8.3 Fenced Reserves Conserving Cheetahs and African Wild Dogs in South Africa
1Carnivore Conservation Programme,
Endangered Wildlife Trust,
Johannesburg, South Africa.
2Current address: Department of Nature Conservation,
Tshwane University of Technology,
Pretoria, South Africa.
marnewickKA@tut.ac.za
South Africa is one of the few countries in Africa where numbers of many large carnivore species are stable and, in some cases, increasing. Much of this success can be attributed to the managed metapopulation approach, which involves the reintroduction and subsequent translocation and management of populations in geographically isolated fenced reserves, between which natural dispersal is highly unlikely. As of 2016, more than 300 cheetahs are being managed in 51 reserves encompassing 10,995 km2 (mean: 195 km2 range: 20–1,000 km2) and nearly 250 African wild dogs in 11 reserves encompassing 5,086 km2 (mean: 216 km2 range: 19–1,000 km2). The reserves are situated across the country within a variety of land tenure systems including state and provincial protected areas and privately owned and community-run game reserves. Most reserves derive income primarily from ecotourism.
Each reserve forms part of the national network. Animals are moved between reserves to maintain the genetic integrity and demographic balance of individual subpopulations, but also to minimise direct management in the long term. Translocations are planned to mimic natural processes as far as possible but, due to the intricacies involved in managing animals between several reserves, this is not always possible. For wild dogs, small groups of unrelated adult males and females are artificially bonded to form packs, which mimics natural pack formation in the wild. For cheetahs, sub-adults are removed once they disperse from their maternal range. The animals are generally immobilised in the field and transported awake in crates on vehicles to their new reserves. Soft releases (Section 11.2.1) are preferred: these involve the animals being kept in temporary holding bomas of approximately 1 ha in size for about three months. The formation of artificial social groups is also done during this period. Intensive post-release monitoring is done at intervals reliant on reserve resources, but daily monitoring is recommended. The success rate of reintroductions has been high and, for wild dogs, has been strongly linked to the social cohesion of released groups (Marneweck et al., 2019), and the integrity of perimeter fences (Gusset et al., 2008).
This highly collaborative process involves multiple stakeholders, including conservation NGOs, provincial government conservation departments, private reserve owners and managers, researchers, local communities, and tourists. Effective and responsible population management tools help to prevent local populations growing too large or too small, and best practice guidelines ensure the ethical handling and management of animals. Individual reserves are responsible for providing infrastructure and other requirements including managing sustainable prey populations, perimeter fences, bomas and post release monitoring, as well as ensuring that a management plan is in place and adhered to. In many cases, students or volunteer organisations conduct post-release monitoring. National, high-level management is coordinated by the Endangered Wildlife Trust (EWT) and is funded through donations from corporations, individual philanthropists, conservation trusts, and foundations.
The managed metapopulation approach to carnivore conservation has increased the number and distribution of both cheetahs and African wild dogs in South Africa and built technical capacity in the country for metapopulation management (Davies-Mostert and Gusset, 2013), which has also been applied to species, such as lions, elephants, and black rhinoceros (Diceros bicornis, CR). Opportunities abound in other countries to use lessons learned in South Africa for the recolonisation of other areas where large mammals have been locally or regionally extirpated. Additionally, projected human population expansion, and the habitat fragmentation that comes with it, means that this approach is likely to become an indispensable tool in maintaining the viability of populations in disconnected landscapes.
8.8 Is De-extinction a Solution?
One of the more interesting conservation debates to have emerged in recent years involve efforts to reverse extinction. This field, known as de-extinction or resurrection biology aims to revive extinct species, and eventually to reintroduce viable populations to their original locations (Seddon, 2017). One possible method, called “breeding back”, aims to produce individuals genetically similar to an extinct species by selective breeding of extant species that carry genetic material of their extinct relatives. This is the main method currently being used to revive the aurochs (Bos primigenius, EX), the ancestor of today’s domestic cattle (Stokstad, 2015). Other “breeding back” projects place less emphasis on genetics and more on morphology, by selectively breeding individuals with certain traits to produce individuals that visually appear similar to the extinct species. Such is the case at The Quagga Project, where selectively breeding of plains zebras (Equus quagga, NT) with quagga-like characteristics (reduced striping and brown hues) are resulting in animals (Figure 8.11) that look increasingly like extinct quaggas (Harley et al., 2009).
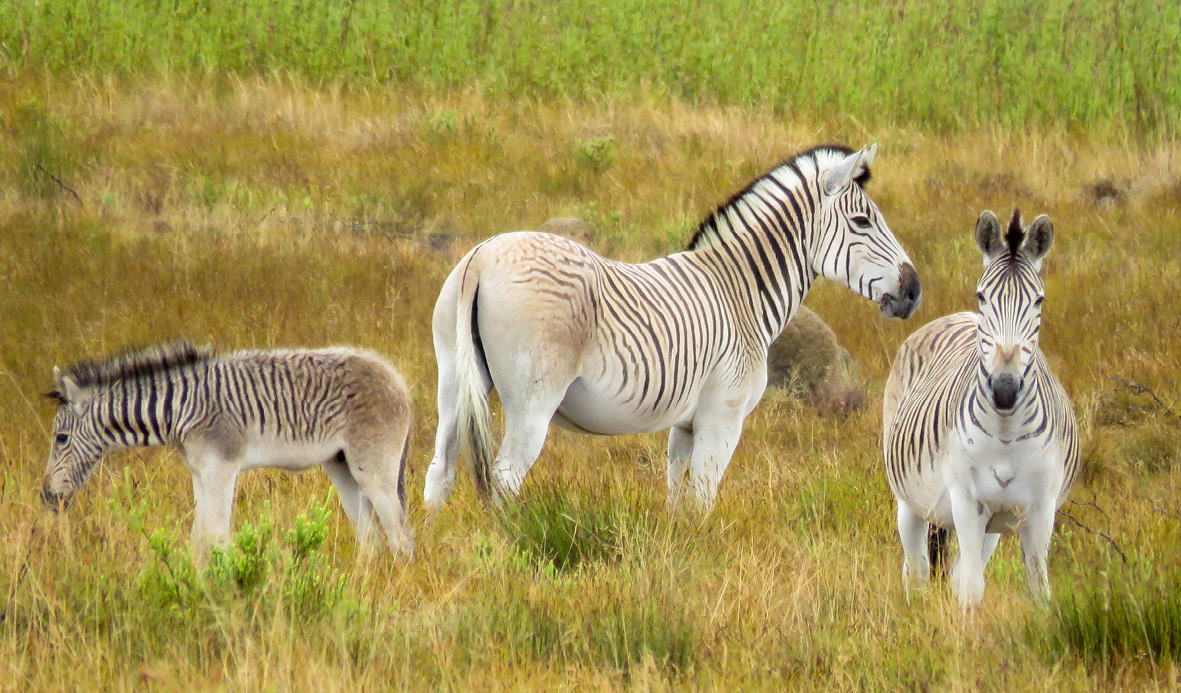
Figure 8.11 Not extinct anymore? A selective breeding programme in South Africa has raised several plain’s zebras that closely resemble the extinct quagga. The progress from one generation to the next can be seen in this photo, with the adults showing reduced striping, and the foal showing brown hues. Photograph by The Quagga Project, CC BY 4.0.
The second popular method used for de-extinction is cloning. This involves the transfer of viable genetic material from an extinct species to the eggs (or embryo) of a closely related surrogate mother, who will hopefully give birth to an individual of the extinct species. Cloning has been used in selective breeding of livestock for many years, and plans are also currently underway to use cloning to prevent the extinction of highly threatened species such as the northern white rhinoceros (see Box 11.4). Despite the promise that cloning offers for reviving extant and recently extinct species, cloning species that went extinct many years ago has been more challenging. So far, attempts to clone Spain’s Pyrenean ibex (Capra pyrenaica pyrenaica, EX) and Australia’s gastric-brooding frog (Rheobatrachus silus, EX) have produced individuals that lived for only a few minutes (Ogden, 2014).
Despite the progress made, de-extinction is one of the most controversial and polarising debates to emerge among conservation biologists in recent years. Proponents of de-extinction hope that the early work described above paves the way for the resurrection of extinct species once the threats that drove them to extinction have been managed. Many resurrection biologists have even started establishing banks where genetic material of threatened species is cryopreserved for future use. They also hope that their work will inspire more people to be interested in science in general and especially in conservation. Protected areas with extinct species may even draw tourists that can fund conservation projects, while reintroductions of once-extinct species can revive lost ecosystem services.
Bringing a species back from extinction is, however, highly controversial for several reasons. First, there is the argument that the limited funds available for conservation are better spent on species currently facing extinction rather than on projects with possibly insurmountable technical challenges. Others argue that there is no point in spending millions of dollars to bring back an extinct species if we cannot even solve the extinctions drivers that caused the demise of the extinct species in the first place. Conservationists also wonder how the issues facing small populations will be managed, especially early in the process. Many believe that these small compromised populations will simply occupy valuable space in zoos and protected areas that can be better used for protecting extant species. There are also major misgivings about whether the resurrected species will fill the same ecosystem function as before since they may behave differently; in fact, some worry that unpredictable behaviours may introduce new harmful threats to ecosystems. Many conservationists are also worried that the public’s concern for species currently threatened may fade if there is a perception that we can simply revive the species after the last individual died. Lastly, ethical questions are frequently raised about humans essentially trying to “play God” with these “vanity projects”, and the possibility that this entire field will undermine one of the most important foundations of conservation biology—that we need to act now because extinction is forever. Clearly there are some advantages, but also disadvantages, to de-extinction. Most importantly, much research still needs to happen for this to be a viable idea.
8.9 Summary
- The rates of species extinction are currently 1,000 times greater than natural background levels; this may soon increase to 10,000 times. Over 99% of modern extinctions can be attributed to human activity. These extinctions are leading to the rapid loss of ecosystem services.
- The IUCN has developed quantitative criteria that assign species to nine conservation categories based on their risk of extinction. Species that are Extinct in the Wild (EW), Critically Endangered (CR), Endangered (EN), and Vulnerable (VU) are officially considered “threatened with extinction”. The five other categories are Extinct (EX), Near Threatened (NT), Least Concern (LC), Data Deficient (DD), and Not Evaluated (NE).
- Species with the following characteristics are particularly vulnerable to extinction: species with small populations, species with declining populations, species with restricted distribution ranges, species with one or only a few small populations, species that are exploited by people, and species with critical symbiotic relationships.
- Small populations are at high risk of extinction because they are vulnerable to several deleterious genetic factors, as well as demographic and environmental stochasticity. Such populations often require intensive management to prevent them from becoming a victim of an extinction vortex.
- De-extinction as a scientific field aims to revive extinct species using methods such as selective breeding and cloning. But this practice is controversial, and not practical with current technology.
8.10 Topics for Discussion
- Why should you, or anyone else, be concerned if a species becomes extirpated (also known as locally extinct)? How does this concern compare to when a species becomes globally extinct?
- Use the IUCN Red List (http://www.iucnredlist.org) to identify one species in your country that is currently threatened with extinction. How might this species be affected by the various challenges facing small populations? Consider genetic, physiological, behavioural, and ecological factors, as appropriate.
- Think of an imaginary animal that was recently discovered. Imagine this animal is also threatened with extinction. Name three characteristics that make your imaginary animal vulnerable to its threats. Now discuss some steps that can be implemented to ensure that your animal will continue to survive.
- A herd of 80 rhinoceros have been moved from South Africa to Australia to “save the species” because “there is no safe place in Africa for rhinos today” (see Hayward et al., 2017). What do you think of this plan? Do you think the project will be successful? What are the main opportunities and challenges? Once you’re done answering the question read Lundgren et al. (2017) and decide if you still feel the same.
8.11 Suggested Readings
Bonebrake, T.C., F. Guo, C. Dingle, et al. 2019. Integrating proximal and horizon threats to biodiversity for conservation. Trends in Ecology and Evolution 34: in press. https://doi.org/10.1016/j.tree.2019.04.001 Coordination is critical when combatting multiple threats.
Caughley, G. 1994. Directions in conservation biology. Journal of Animal Ecology 63: 215–24. https://doi.org/10.2307/5542 The classic overview of the challenges faced by small and declining populations.
Ceballos, G., P.R. Ehrlich, and R. Dirzo. 2017. Biological annihilation via the ongoing sixth mass extinction signaled by vertebrate population losses and declines. Proceedings of the National Academy of Sciences 114: E6089–E6096. https://doi.org/10.1073/pnas.1704949114 Extinctions by the numbers.
IBPES. 2019. Nature’s dangerous decline ‘unprecedented’: Species extinction rates accelerating. IBPES media release. https://www.ipbes.net/news/Media-Release-Global-Assessment We are in the midst of a biodiversity crisis.
IUCN. 2012. IUCN Red List Categories and Criteria: v. 3.1 (Gland: IUCN). https://portals.iucn.org/library/sites/library/files/documents/RL-2001-001-2nd.pdf A summary of the IUCN Red List classifications.
Jones, H.P. 2010. Seabird islands take mere decades to recover following rat eradication. Ecological Applications 20: 2075–80. https://doi.org/10.1890/10-0118.1 Control of invasive species can be an effective method to achieve recovery of threatened species.
Koh, L.P., R.R. Dunn, N.S. Sodhi, et al. 2004. Species coextinctions and the biodiversity crisis. Science 305: 1632–34. https://doi.org/10.1126/science.1101101 Thousands of species face extinction due to the decoupling of important symbiotic relationships.
McClenachan, L., A.B. Cooper, K.E. Carpenter, et al. 2012. Extinction risk and bottlenecks in the conservation of charismatic marine species. Conservation Letters 5: 73–80. https://doi.org/10.1111/j.1755-263X.2011.00206.x Most marine species have not been evaluated for their risk of extinction.
Stearns, B.P., and S.C. Stearns. 2010. Still watching, from the edge of extinction. BioScience 60: 141–46. https://doi.org/10.1525/bio.2010.60.2.8 Many threatened species increasingly rely on human actions to avoid extinction.
Tranquilli, S., M. Abedi-Lartey, F. Amsini, et al. 2012. Lack of conservation effort rapidly increases African great ape extinction risk. Conservation Letters 5: 48–55. https://doi.org/10.1111/j.1755-263X.2011.00211.x Neglecting species in conservation need increases extinction risk.
Bibliography
Andreotti, S., S. Heyden, R. Henriques, et al. 2016. New insights into the evolutionary history of white sharks, Carcharodon carcharias. Journal of Biogeography 43: 328–39. https://doi.org/10.1111/jbi.12641
Balon, E.K., M.N. Bruton, and H. Fricke. 1988. A fiftieth anniversary reflection on the living coelacanth, Latimeria chalumnae: Some new interpretations of its natural history and conservation status. Environmental Biology of Fishes 23: 241–80. https://doi.org/10.1007/BF00005238
Barnosky, A.D., N. Matzke, S. Tomiya, et al. 2011. Has the Earth’s sixth mass extinction already arrived? Nature 471: 51–57. https://doi.org/10.1038/nature09678
Barnosky, A.D., P.L. Koch, R.S. Feranec, et al. 2004. Assessing the causes of late Pleistocene extinctions on the continents. Science 306: 70–75. https://doi.org/10.1126/science.1101476
Barraclough, D.A. 2006. Bushels of bots. Natural History 115: 18–21.
Bartlett, L.J., D.R. Williams, G.W. Prescott, et al. 2015. Robustness despite uncertainty: Regional climate data reveal the dominant role of humans in explaining global extinctions of Late Quaternary megafauna. Ecography 39: 152–61. https://doi.org/10.1111/ecog.01566
Bauer, H., C. Packer, P.F. Funston, et al. 2015. Panthera leo. The IUCN Red List of Threatened Species 2015: e.T15951A79929984. https://doi.org/10.2305/IUCN.UK.2016-3.RLTS.T15951A107265605.en
Bazelet, C., and P. Naskrecki. 2014. Aroegas nigroornatus. The IUCN Red List of Threatened Species 2014: e.T20639917A56180093. https://doi.org/10.2305/IUCN.UK.2014-1.RLTS.T20639917A56180093.en
BirdLife International 2018a. Crithagra concolor. The IUCN Red List of Threatened Species 2018: e.T22720310A128249895. https://doi.org/10.2305/IUCN.UK.2018-2.RLTS.T22720310A128249 895.en
BirdLife International 2018b. Lanius newtoni. The IUCN Red List of Threatened Species 2018: e.T22705080A131390093. https://doi.org/10.2305/IUCN.UK.2018-2.RLTS.T22705080A131390 093.en
Blackburn, D.C., C. Boix, E. Greenbaum, et al. 2016. The distribution of the Bururi Long-fingered Frog (Cardioglossa cyaneospila, family Arthroleptidae), a poorly known Albertine Rift endemic. Zootaxa 4170: 355–64. http://doi.org/10.11646/zootaxa.4170.2.8
Boschian, G., D. Caramella, D. Saccà, et al. 2019. Are there marrow cavities in Pleistocene elephant limb bones, and was marrow available to early humans? New CT scan results from the site of Castel di Guido (Italy). Quaternary Science Reviews 215 86–97. https://doi.org/10.1016/j.quascirev.2019.05.010
Brito, J.C., S.M. Durant, N. Pettorelli, et al. 2018. Armed conflicts and wildlife decline: Challenges and recommendations for effective conservation policy in the Sahara-Sahel. Conservation Letters 11: e12446. http://doi.org/10.1111/conl.12446
Brook, B.W., and M.J.S. Bowman. 2005. One equation fits overkill: Why allometry underpins both prehistoric and modern body-sized extinctions. Population Ecology 47: 137–41. https://doi.org/10.1007/s10144-005-0213-4
Brooks, T.M., S.L. Pimm, and J.O. Oyugi. 1999. Time lag between deforestation and bird extinction in tropical forest fragments. Conservation Biology 13: 1140–50. https://doi.org/10.1046/j.1523-1739.1999.98341.x
Burt, A.J., J. Gane, I. Olivier, et al. 2016. The history, status and trends of the Endangered Seychelles Magpie-robin Copsychus sechellarum. Bird Conservation International 26: 505–23. https://doi.org/10.1017/S0959270915000404
Callaway, E. 2017. Oldest Homo sapiens fossil claim rewrites our species’ history. Nature News. https://doi.org/10.1038/nature.2017.22114
Caughley, G. 1994. Directions in conservation biology. Journal of Animal Ecology 63: 215–24. https://doi.org/10.2307/5542
Ceballos, G., P.R. Ehrlich, A.D. Barnosky, et al. 2015. Accelerated modern human-induced species losses: Entering the sixth mass extinction. Science Advances 1: e1400253. https://doi.org/10.1126/sciadv.1400253
Ceballos, G., P.R. Ehrlich, and R. Dirzo. 2017. Biological annihilation via the ongoing sixth mass extinction signaled by vertebrate population losses and declines. Proceedings of the National Academy of Sciences 114: E6089–E6096. https://doi.org/10.1073/pnas.1704949114
Chakona, A, and S. Kubheka. 2018. Pseudobarbus quathlambae. The IUCN Red List of Threatened Species 2018: e.T18475A100171498. http://doi.org/10.2305/IUCN.UK.2018-2.RLTS.T18475A1 00171498.en
Channing, A., K.S. Finlow-Bates, S.E. Haarklau, et al. 2006. The biology and recent history of the Critically Endangered Kihansi Spray Toad Nectophrynoides asperginis in Tanzania. Journal of East African Natural History 95: 117–38. https://doi.org/10.2982/0012-8317(2006)95[117:TBARHO]2.0.CO;2
Chiyo, P.I., V. Obanda, and D.K. Korir. 2015. Illegal tusk harvest and the decline of tusk size in the African elephant. Ecology and Evolution 5: 5216–29. https://doi.org/10.1002/ece3.1769
Clarke, G.P., N.D. Burgess, F.M. Mbago, et al. 2011. Two ‘extinct’ trees rediscovered near Kilwa, Tanzania. Journal of East African Natural History 100: 133–40. https://doi.org/10.2982/028.100.0109
Contu, S. 2013. Cyperus chionocephalus. The IUCN Red List of Threatened Species 2013: e.T44393328A44490119. http://doi.org/10.2305/IUCN.UK.2013-1.RLTS.T44393328A44490119.en
Courchamp, F., T. Clutton-Brock, and B. Grenfell. 2000. Multipack dynamics and the Allee effect in the African wild dog, Lycaon pictus. Animal Conservation 3: 277–85. https://doi.org/10.1111/j.1469-1795.2000.tb00113.x
Cowlishaw, G. 1999. Predicting the pattern of decline of African primate diversity: An extinction debt from historical deforestation. Conservation Biology 13: 1183–93. https://doi.org/10.1046/j.1523-1739.1999.98433.x
Cronk, Q. 2016. Plant extinctions take time. Science 353: 446–47. https://doi.org/10.1126/science.aag1794
Cumming, D.H.M. 2007. Of elephants, predators, and plants in protected areas: A case of classic trophic cascades. Paper presented in Symposium: Sharing the range: Elephants, people and biological conservation in Africa. SCB Annual Meeting, 1–5 July, Port Elizabeth, South Africa.
Dalton, D.L., E. Vermaak, H.A. Smit-Robinson, et al. 2016. Lack of diversity at innate immunity Toll-like receptor genes in the Critically Endangered White-winged Flufftail (Sarothrura ayresi). Scientific Reports 6: 36757. https://doi.org/10.1038/srep36757
Davies-Mostert H.T., and M. Gusset. 2013. Restoring African wild dogs in South Africa: A managed metapopulation approach. WAZA Magazine 14: 41–44
de Vos, J.M., L.N. Joppa, J.L. Gittleman, P.R. Stephens, et al. 2015. Estimating the normal background rate of species extinction. Conservation Biology 29: 452–62. https://doi.org/10.1111/cobi.12380
Durant, S.M., T. Wacher, S. Bashir, et al. 2013. Fiddling in biodiversity hotspots while deserts burn? Collapse of the Sahara’s megafauna. Diversity and Distributions 20: 114–22. https://doi.org/10.1111/ddi.12157
Durant, S., N. Mitchell, A. Ipavec, et al. 2017. The global decline of cheetah Acinonyx jubatus and what it means for conservation. Proceedings of the National Academy of Sciences 114: 528–33. https://doi.org/10.1073/pnas.1611122114
Entwistle, A.C., and J. Juma. 2016. Pteropus voeltzkowi. The IUCN Red List of Threatened Species 2016: e.T18768A22089205. http://doi.org/10.2305/IUCN.UK.2016-1.RLTS.T18768A22089205.en
Fagan, W.F., and E.E. Holmes. 2006. Quantifying the extinction vortex. Ecology Letters 9: 51–60. https://doi.org/10.1111/j.1461-0248.2005.00845.x
Faith, J.T., J. Rowan, A. Du, et al. 2018. Plio-Pleistocene decline of Africa’s megaherbivores: No evidence for ancient hominin impacts. Science 362: 938–41. https://doi.org/10.1126/science.aau2728
Feranec, R.S. 2004. Geographic variation in the diet of hypsodont herbivores from the Rancholabrean of Florida. Palaeogeography, Palaeoclimatology, Palaeoecology 207: 359369. https://doi.org/10.1016/j.palaeo.2003.09.031
Frankham, R., J.D. Ballou, M.D.B. Eldridge, et al. 2011. Predicting the probability of outbreeding depression. Conservation Biology 25: 465–75. https://doi.org/10.1111/j.1523-1739.2011.01662.x
Gibert, P., R.B. Huey, and G.W. Gilchrist. 2001. Locomotor performance of Drosophila melanogaster: interactions among developmental and adult temperatures, age, and geography. Evolution 55: 205–09. https://doi.org/10.1111/j.0014-3820.2001.tb01286.x
Gilpin, M.E., and M.E. Soulé. 1986. Minimum viable populations: Processes of species extinction. In: The Science of Scarcity and Diversity, ed. by M.E. Soulé (Sunderland: Sinauer).
Goldschmidt, T., F. Witte, and J. Wanink. 1993. Cascading effects of the introduced Nile perch on the detritivorous/phytoplanktivorous species in the sublittoral areas of Lake Victoria. Conservation Biology 7: 686–700. https://doi.org/10.1046/j.1523-1739.1993.07030686.x
Grobler, J.P., I. Rushworth, J.S. Brink, et al. 2011. Management of hybridization in an endemic species: Decision making in the face of imperfect information in the case of the black wildebeest—Connochaetes gnou. European Journal of Wildlife Research 57: 997–1006. https://doi.org/10.1007/s10344-011-0567-1
Guerrant, E.O. 1992. Genetic and demographic considerations in the sampling and reintroduction of rare plants. In: Conservation Biology: The Theory and Practice of Nature Conservation, Preservation and Management, ed. by P.L. Fiedler and S.K. Jain (New York: Springer). https://doi.org/10.1007/978-1-4684-6426-9
Gusset, M., S.J. Ryan, M. Hofmeyr, et al. 2008. Efforts going to the dogs? Evaluating attempts to re‐introduce endangered wild dogs in South Africa. Journal of Applied Ecology 45: 100–08. https://doi.org/10.1111/j.1365-2664.2007.01357.x
Halley, J.M., N. Monokrousos, A.D. Mazaris, et al. 2016. Dynamics of extinction debt across five taxonomic groups. Nature Communications 7: 12283. https://doi.org/10.1038/ncomms12283
Harley, E.H., M.H. Knight, C. Lardner, et al. 2009. The Quagga project: Progress over 20 years of selective breeding. South African Journal of Wildlife Research 39: 155–63. https://doi.org/10.3957/056.039.0206
Haynes G. 2018. The evidence for human agency in the Late Pleistocene megafaunal extinctions. In Encyclopedia of the Anthropocene v. 1, ed. by D.A. DellaSala, and M.I. Goldstein (Oxford: Elsevier).
Hayward, M.W., W.J. Ripple, G.I.H. Kerley, et al. 2017. Neocolonial conservation: Is moving rhinos to Australia conservation or intellectual property loss? Conservation Letters 11: e12354. https://doi.org/10.1111/conl.12354
Heber, S., and J.V. Briskie. 2010. Population bottlenecks and increased hatching failure in endangered birds. Conservation Biology 24: 1674–78. https://doi.org/10.1111/j.1523-1739.2010.01553.x
Hutton, J.M. 1987. Incubation temperatures, sex ratios and sex determination in a population of Nile crocodiles (Crocodylus niloticus). Journal of Zoology 211: 143–55. http://doi.org/10.1111/j.1469-7998.1987.tb07458.x
IUCN. 2012. IUCN Red List categories and criteria: v. 3.1 (Gland: IUCN). https://portals.iucn.org/library/sites/library/files/documents/RL-2001-001-2nd.pdf
IUCN. 2017. Guidelines for using the IUCN Red List categories and criteria, v. 13 (Gland: IUCN). http://nc.iucnredlist.org/redlist/content/attachment_files/RedListGuidelines.pdf
IUCN. 2019. The IUCN Red List of Threatened Species. http://www.iucnredlist.org
Janzen, D.H. 1983. The Pleistocene hunters had help. American Naturalist 121: 598–99. https://doi.org/10.1086/284088
Johnson, C.N. 2009. Ecological consequences of Late Quaternary extinctions of megafauna. Proceedings of the Royal Society of London B 276: 2509–19. https://doi.org/10.1098/rspb.2008.1921
Kalinowski, S.T., P.W. Hedrick, and P.S. Miller. 2000. Inbreeding depression in the Speke’s gazelle captive breeding program. Conservation Biology 14: 1375–84. https://doi.org/10.1046/j.1523-1739.2000.98209.x
Keith, D.A., J.P. Rodríguez, K.M. Rodríguez-Clark, et al. 2013. Scientific foundations for an IUCN Red List of Ecosystems. PLoS ONE 8: e62111. https://doi.org/10.1371/journal.pone.0062111
Kerley, H.I.H., R. Sims-Castley, A.F. Boshoff, et al. 2009. Extinction of the blue antelope Hippotragus leucophaeus: Modelling predicts non-viable global population size as the primary driver. Biodiversity and Conservation 18: 3235. https://doi.org/10.1007/s10531-009-9639-x
Koh, L.P., R.R. Dunn, N.S. Sodhi, et al. 2004. Species coextinctions and the biodiversity crisis. Science 305: 1632–34. https://doi.org/10.1126/science.1101101
Krajick, K. 2003. Defusing Africa’s killer lakes. Smithsonian Magazine 34: 46–55. https://www.smithsonianmag.com/science-nature/defusing-africas-killer-lakes-88765263
Leimu, R., and M. Fischer. 2010. Between-population outbreeding affects plant defence. PLoS ONE 5: e12614. https://doi.org/10.1371%2Fjournal.pone.0012614
Lombardo, M.P., and R.O Deaner. 2018. Born to throw: The ecological causes that shaped the evolution of throwing in humans. Quarterly Review of Biology 93: 1–16. https://doi.org/10.1086/696721
Lundgren, E.J., D. Ramp, W.J. Ripple, et al. 2017. Introduced megafauna are rewilding the Anthropocene. Ecography 41: 857–66. https://doi.org/10.1111/ecog.03430
Marneweck, C., P.A. Becker, G. Beverley, et al. 2019. Factors affecting the success of artificial pack formation in an endangered, social carnivore: The African wild dog. Animal Conservation 22: in press. https://doi.org/10.1111/acv.12490
Maron, D.F. 2018. Under poaching pressure, elephants are evolving to lose their tusks. National Geographic. https://on.natgeo.com/2z9mE1x
Meffe, G.C., and C.R. Carroll. 1997. Principles of Conservation Biology (Sunderland: Sinauer).
Minnaar, A., L. van Schalkwyk, and S. Kader. 2018. The difficulties in policing and combatting of a maritime crime: The case of Abalone poaching along South Africa’s coastline. Journal of the Indian Ocean Region 14: 71–87. https://doi.org/10.1080/19480881.2018.1421448
Ogden, L.E. 2014. Extinction is forever… or is it? BioScience 64: 469–75. https://doi.org/10.1093/biosci/biu063
Okubamichael, D.Y., S. Jack, J. de Wet Bösenberg, et al. 2016. Repeat photography confirms alarming decline in South African cycads. Biodiversity and Conservation 25: 2153–70. http://doi.org/10.1007/s10531-016-1183-x
OpenStax. 2019. Biology 2nd ed. OpenStax CNX. https://openstax.org/details/books/biology-2e
Owen‐Smith, N., G.J. Chirima, V. Macandza, et al. 2012. Shrinking sable antelope numbers in Kruger National Park: What is suppressing population recovery? Animal Conservation 15: 195–204. https://doi.org/10.1111/j.1469-1795.2011.00504.x
Pimm, S.L., C.N. Jenkins, R. Abell, et al. 2014. The biodiversity of species and their rates of extinction, distribution, and protection. Science 344: 1246752. https://doi.org/10.1126/science.1246752
Pringle, R.M. 2005. The origins of the Nile perch in Lake Victoria. BioScience 55: 780–87. https://doi.org/10.1641/0006-3568(2005)055[0780:TOOTNP]2.0.CO;2
Rall, J.L., and T. Sephaka. 2008. Re-evaluation of the relevance to construct barriers as in-situ conservation measures for the protection of the Maloti minnow in the Senqunyane Catchment (Florida: ECOSUN Environmental Consultants).
Rebaudo, F., and V.‐B. Rabhi. 2018. Modeling temperature‐dependent development rate and phenology in insects: Review of major developments, challenges, and future directions. Entomologia Experimentalis et Applicata 166: 607–17. https://doi.org/10.1111/eea.12693
Rebelo, A.G. 1992. Red Data Book species in the Cape Floristic Region: Threats, priorities and target species. Transactions of the Royal Society of South Africa 48: 55–86. https://doi.org/10.1080/00359199209520256
Ripple, W.J., and B. Van Valkenburgh. 2010. Linking top-down forces to the Pleistocene megafaunal extinctions. BioScience 60: 516–26. https://doi.org/10.1525/bio.2010.60.7.7
Schleuning, M., and D. Matthies. 2009. Habitat change and plant demography: Assessing the extinction risk of a formerly common grassland perennial. Conservation Biology 23: 174–83. https://doi.org/10.1111/j.1523-1739.2008.01054.x
Seddon, P.J. 2017. The ecology of de-extinction. Functional Ecology 31: 992–95. https://doi.org/10.1111/1365-2435.12856
Shelton, J.M., B.M. Clark, T. Sephaka, et al. 2017. Population crash in Lesotho’s endemic Maloti minnow Pseudobarbus quathlambae following invasion by translocated smallmouth yellowfish Labeobarbus aeneus. Aquatic Conservation 65–77. https://doi.org/10.1002/aqc.2633
Skelton, P.H., J.L. Rall, E.R Swartz, et al. 2001. Maloti minnow conservation project, LHDA Report. 1041 (Grahamstown: JLB Smith Institute of Ichthyology).
Smith, F.A., R.E. Elliott Smith, S.K. Lyons, et al. 2019. The accelerating influence of humans on mammalian macroecological patterns over the late Quaternary. Quaternary Science Reviews 211: 1–16. https://doi.org/10.1016/j.quascirev.2019.02.031
Steyn, G., J.L. Rall, V. Rall, et al. 1996. Fish. In: Baseline biology survey and reserve development: Phase 1B. v. 3-Fauna Lesotho, LHDA Report 1008, ed. by AfriDev Consultants (Maseru: Lesotho Highlands Development Authority).
Stokstad, E. 2015. Bringing back the aurochs. Science 350: 1144–47. https://doi.org/10.1126/science.350.6265.1144
Surovell, T., N. Waguespack, and P.J. Brintingham. 2005. Global archaeological evidence for proboscidians overkill. Proceedings of the National Academy of Science 102: 6321–26. https://doi.org/10.1073/pnas.0501947102
Tallman, G., J. Zhu, B.T. Mawson, et al. 1997. Induction of CAM in Mesembryanthemum crystallinum abolishes the stomatal response to blue light and light-dependent zeaxanthin formation in guard cell chloroplasts. Plant and Cell Physiology 38: 236–42. https://doi.org/10.1093/oxfordjournals.pcp.a029158
Trinkel, M., D. Cooper, C. Packer, et al. 2011. Inbreeding depression increases susceptibility to bovine tuberculosis in lions: An experimental test using an inbred-outbred contrast through translocation. Journal of Wildlife Diseases 47: 494–500. https://doi.org/10.7589/0090-3558-47.3.494
Van Valkenburgh, B., M.W. Hayward, W.J. Ripple, et al. 2016. The impact of large terrestrial carnivores on Pleistocene ecosystems. Proceedings of the National Academy of Sciences 113: 862–67. https://doi.org/10.1073/pnas.1502554112
Waters, C.N., J. Zalasiewicz, C. Summerhayes, et al. 2016. The Anthropocene is functionally and stratigraphically distinct from the Holocene. Science 351: aad2622. https://doi.org/10.1126/science.aad2622
Werdelin, L., and M.E. Lewis. 2013. Temporal change in functional richness and evenness in the eastern African Plio-Pleistocene carnivoran guild. PLoS ONE 8: e57944. https://doi.org/10.1371/journal.pone.0057944
Whitehouse, A.M. 2002. Tusklessness in the elephant population of the Addo Elephant National Park, South Africa. Journal of Zoology 257: 249–54. https://doi.org/10.1017/S0952836902000845
Witte, F., T. Goldschmidt, P.C. Goudswaard, et al. 1991. Species extinction and concomitant ecological changes in Lake Victoria. Netherlands Journal of Zoology 42: 214–32. https://doi.org/10.1163/156854291X00298
Wright, S. 1931. Evolution in Mendelian populations. Genetics 16: 97–159. http://www.ncbi.nlm.nih.gov/pubmed/17246615
Xue, Y., J. Prado-Martinez, P.H. Sudmant, et al. 2015. Mountain gorilla genomes reveal the impact of long-term population decline and inbreeding. Science 348: 242–45. https://doi.org/10.1126/science.aaa3952