7. The Natural History of Medicinal Flies
© 2022 Michelle L. Harvey, CC BY-NC 4.0 https://doi.org/10.11647/OBP.0300.07
When flies are used for therapeutic purposes to treat wounds (maggot therapy), they may be referred to as medicinal flies. Species that have been used for maggot therapy or which are likely candidates for maggot therapy generally belong to the family Calliphoridae, commonly known as blowflies. These flies have ecological relationships, life-history patterns, physiologies, and nutritional requirements that help them exploit cadavers as well as living bodies. The same adaptations can also be harnessed to treat non-healing necrotic wounds. This chapter first introduces the general features of dipteran diversity, morphology, and biology before a closer examination of the family Calliphoridae.
Introduction
The insect order Diptera is taxonomically and biologically diverse, with in excess of 160,000 described species across 150 families, including a few thousand with medical and veterinary significance [1]. The immense success of the Diptera is evident from their diversity, abundance and virtual omnipresence across habitats, and is a product of highly variable morphology, behaviour, and reproductive biology. Many fly species are closely associated with human activities and settlements. The most thoroughly studied dipteran species comprise those with detrimental effects on society, whether for their biting habits, disease transmission, or pure nuisance value. A key family recognised for their medical significance is the Calliphoridae or blowflies.
When flies are used for therapeutic purposes to treat wounds (maggot therapy), they may be referred to as medicinal flies. Chapter 11 explains the research process leading to the establishment of a new medicinal fly species [2]. Species that have been used for maggot therapy or which are likely candidates for maggot therapy generally belong to the Calliphoridae, with the exception of Wohlfahrtia nuba (Sarcophagidae) [3] and Musca domestica (Muscidae). The latter species was successfully used by Chinese physicians to treat a catastrophic hot-crush injury [4]. Blowflies have ecological relationships, life-history patterns, physiologies, and nutritional requirements that help them exploit cadavers as well as living bodies. The same adaptations can also be harnessed to treat non-healing necrotic wounds.
This chapter will first introduce the general features of dipteran diversity, morphology, and biology before a closer examination of the family Calliphoridae.
Life Histories
Broadly divided into two suborders, the lower Diptera and the Brachycera, the order Diptera is highly diverse in morphology, biology, and behaviour. Diverse feeding strategies and optimal microenvironments enable dipterans to invade a large range of habitats. Their holometabolous life history further contributes to their taxonomic and numerical abundance. Holometabolous insects exhibit a complete metamorphosis, a strategy that sees larvae and adults occupy often disparate habitats with differing feeding preferences, allowing immatures and adults to avoid competition for a single food substrate [5]. Metamorphosis from larva to adult generally takes place within an immobile pupa, with often complete remodelling of external and internal morphological structures, including digestive and reproductive systems.
Morphology
Morphology of the Diptera
Morphology in the Diptera is highly variable and reflective of the diversity of taxa present in the order [6–8]. The Diptera includes such divergent taxa as fruit flies, mosquitoes, blowflies, sand flies, bee flies, crane flies and midges, and their individual life histories, habitats, feeding and reproductive specialisations necessitate adaptation of morphology to adequately support their respective biology.
Dipteran adults are nearly all characterised by a single pair of functional, mesothoracic forewings and modified, club-like, metathoracic hindwings known as halteres, used largely for balancing and stabilisation [6], but some may be apterous (wingless). Their mobile head bears large compound eyes equipped for strong sight in active flight, and mouthparts often modified into a proboscis or sucking structure and directed ventrally beneath the insect [1]. Mouthpart organisation may be highly modified in taxa equipped for biting and/or blood feeding, with adaptation for rasping, sawing, tearing and injection of mouthparts into the skin all observed in blood feeders. Antennae may be variable and exhibit sexual dimorphism (variation between the sexes) [6]. Legs always bear five tarsomeres and adult genitalia may differ greatly between taxa, which allows differentiation between species.
Larvae are apodous (lacking true legs), but prolegs may be present [6]. The larval form may be variously modified for aquatic habitats in some families (e.g. Culicidae, Simuliidae). Head morphology varies from a sclerotised head capsule in some taxa through to acephaly (no head capsule) and an internal skeleton in others. Larvae lack true articulated mandibles (adecticous), and may metamorphose in a cocoon with appendages glued to the body wall (obtect pupa) or without forming a cocoon and with free appendages (exarate pupa) [1]. A sub-group of the exarate pupae are coarctate, meaning the pupa is contained within the hardened cuticle of the last larval instar and thus retains physical characteristics of the last larval form. This includes the Calliphoridae or blowflies, the subject of this chapter.
Suborder Morphology
The lower Diptera tend to be more delicate, including the mosquitoes, crane flies and midges, with long, slender antennae, and some taxa with aquatic larvae. Larvae of the lower Diptera are usually eucephalic (with a complete head capsule) [6]. The Brachycera are recognised as having more robust adult flies, with shorter, aristate antennae. A characteristic of the cyclorrhaphan flies (a grouping within the Brachycera and focus of this chapter) is the presence of a ptilinum. This is an eversible, bladder-like structure situated between the eyes of the adult [6], used to rupture the pupal casing and allow the emergence of the adult. Following eclosion of the adult, the ptilinum is retracted but evidence of its former presence and purpose is retained in the form of the ptilinal fissure. The bulk of the medically significant Diptera are found within the Brachycera (Table 7.1). The focus of the remainder of this chapter will be the family Calliphoridae.
Table 7.1 Key dipteran taxa recognised for medical and/or veterinary significance.
Suborder |
Taxon |
Family |
Synanthropic Nature |
Lower Diptera |
Mosquitoes |
Culicidae |
Nuisance value from biting; disease transmission (malaria, dengue, yellow fever, Ross River Fever, various forms of Encephalitis, filariasis) |
Black flies |
Simuliidae |
Nuisance value from biting; vector for transmission of onchocerciasis (river blindness) |
|
Biting Midges |
Ceratopogonidae |
Nuisance value from biting |
|
Brachycera |
Tsetse flies |
Glossinidae |
Myiasis; transmission of trypanosomiasis (sleeping sickness) |
Bot flies |
Oestridae |
Myiasis (including humans) |
|
Soldier flies |
Stratiomyidae |
Nuisance value; mechanical vectors of disease; forensic use |
|
Louse flies |
Hippoboscidae |
Obligate parasites of vertebrates |
|
Horse flies |
Tabanidae |
Biting nuisance, plus potential vectors of blood-borne disease |
|
House/bush flies |
Muscidae |
Nuisance value, plus biting in some taxa; forensic use |
|
Flesh flies |
Sarcophagidae |
Nuisance value; some parasitoids; some involvement in myiasis; forensic use |
|
Blowflies |
Calliphoridae |
Nuisance value; involvement in myiasis; forensic use |
Morphology of the Calliphoridae
Calliphorid adults vary greatly in size and colouration, and this diversity is reflected numerically with close to 1,500 described species [9]. Adults are often shiny with metallic colouring or dusted with a luminescent sheen referred to as pollinosity or pruinosity [10], which is best observed in bright sunlight. Three-segmented horned antennae, with a plumose arista and well developed calypters (lobes at the rear of the forewings) are additional classifying features. Within the family, key distinguishing taxonomic features include wing venation and chaetotaxy (bristle arrangement) [10, 11]. Features such as colouration and size of an individual should be treated cautiously when identifying a species. Individuals of the same species may be larger or smaller or look different in other ways because of geographical separation and adaptation to local conditions, or perhaps because their larvae had greater or lesser access to food.
Eggs are cylindrical in shape with rounded ends, and are pale white or yellow-coloured. The chorion (“shell”) bears a median groove referred to as the plastron, which serves as the respiratory surface of the organism, and is also the point of weakness from which first-instar larvae emerge at hatching [5].
Larvae have a pointed anterior end and a blunt posterior end (Figures 7.1 and 7.2). They are apodous and lack a head capsule, instead housing an internal cephalopharyngeal skeleton that may be visible through their relatively translucent integument. Larvae lack mandibulate mouthparts but the mouth hooks are visibly extended from the head, and are used to loosen and separate food particles and for crawling. The structure of the cephalopharyngeal skeleton and associated sclerites may be of taxonomic value [5, 10]. In terrestrial insects, oxygen exchange occurs using breathing structures called spiracles, and these are borne in two places on calliphorid larvae. A pair of anterior spiracles can be found on the second segment, and a pair of posterior spiracles on the twelfth segment. The anterior spiracles are absent, or at least non-functional, in first-instar larvae [10]. The posterior spiracles are taxonomically important, and their structure may be used to distinguish between the three instars. The first-instars bear simple spiracles with a single simple hole, while the second- and third-instars bear two or three pairs of spiracular slits respectively (Figure 7.1) [7, 10]. The spiracular structure is considerably more reliable for distinguishing the relative development of a larva than the use of larval length, width or weight. The integument is ornamented with spines or scales that circle the insect in bands, and may be used taxonomically. Between each larval instar, a moult occurs, enabling larvae to shed their constrictive integument and continue to feed and increase in size in the next stage.
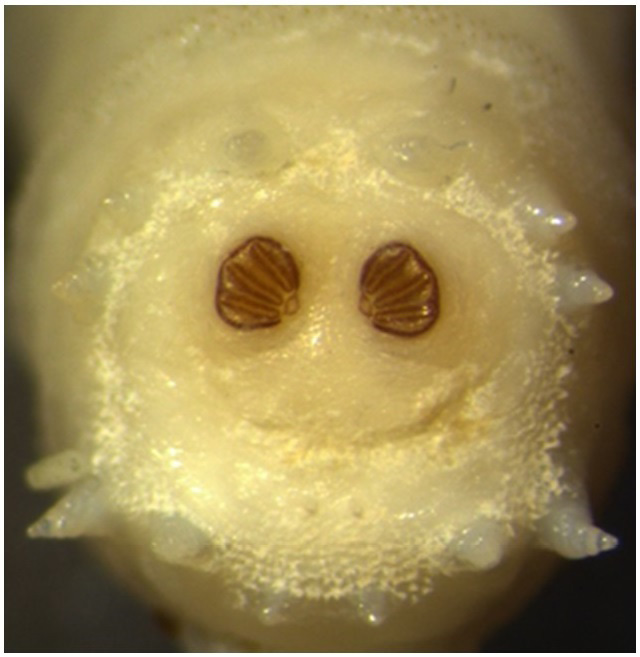
Figure 7.1 Posterior spiracles of third-instar calliphorid larva, showing three pairs of spiracular slits. Photo by M. Harvey, CC BY-NC.
Pupae of calliphorid flies are coarctate, which means that they are retained within the last larval cuticle. At the end of the third-instar phase, this cuticle sclerotises (hardens) and becomes a dark brown colour (Figure 7.2), providing a tough, hydrophobic casing called the puparium. Within the puparium, the larva undergoes extensive remodelling of tissues and organ systems in order to transform into an adult fly (metamorphosis). During this stage, taxonomically important features of the larva are retained on the outside of the puparium [10], and the mouth hooks do not fuse with the puparium.
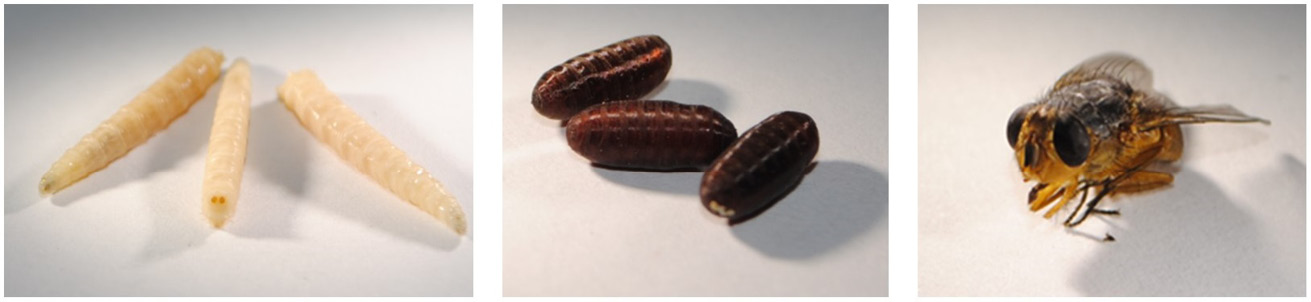
Figure 7.2 Larvae, pupae and adult calliphorid flies. Photos by M. Harvey, CC BY-NC.
Life-cycle and Developmental Traits of Calliphorid Flies
Adults: Attraction and Oviposition Behaviour
Calliphorid females lay eggs in batches of varying size on a nutritionally attractive substrate; frequently a microbially dense, moist surface suitable as a food source for offspring. Individual species have geographical ranges that reflect their species’ biology, generally governed by availability of potential food sources, ambient temperature range, and humidity. When seeking a suitable surface upon which to lay, females are attracted to matter in a particular state of decay, and other factors such as indoor/outdoor/direct sunlight and the presence of fleece/hair on the body surface of the host may also be relevant [5]. How attractive a food source is may also be dependent on microbial contamination of that food. Volatile (easily evaporating) compounds taken from substrate colonised by non-sterile larvae are highly attractive to Lucilia cuprina, compared to volatiles from substrate infested with microbe-free larvae [12].
The females locate a suitable larval food source using highly developed chemoreceptors located on their antennae [5]. These receptors enable the flies to detect a food source from a considerable distance—some studies have indicated attraction of gravid (ready to deposit offspring) females from up to 63km away [13], demonstrating their ability to locate an ephemeral resource with high efficiency. Individual species may exhibit preference for substrate in a particular state of decay, as reflected by its odour, thus the condition of the substrate will determine which species is likely to colonise it. Dry substrate generally lacks appeal [5].
Females may also be attracted to a suitable substrate by pheromones from females of the same species, resulting in an accumulation of eggs in a single spot. Thus, the attraction of a single female to a suitable site may result in large aggregations of eggs [14]. As eggs are laid on top of each other, they protect each other from drying out [5]. The number of eggs laid by a female is thought to vary for different resources depending on the level of competition anticipated and other environmental factors, with the obvious goal being to maximize offspring fitness [15]. It has further been shown that female flies may be attracted by interkingdom bacterial swarming which signals the presence of a suitable food source. For example, swarming Proteus mirabilis attract female greenbottle blowflies (Lucilia sericata) [16].
Not all calliphorid fly species lay eggs. Indeed, there is a bewildering variety of reproductive strategies. While some (oviparous) species lay eggs directly on the food source in batches up to several hundred per fly [17], other species, particularly those found in more extreme climates, may be larviparous and deposit live larvae or ovoviviparous and deposit live larvae ensheathed in a chorion that hatch almost immediately on deposition. Such species exhibit lower batch sizes due to increased maternal investment, but egg desiccation is avoided and larvae can immediately commence feeding. This allows species to capitalise quickly on the presence of an ephemeral and high-value resource, thus providing a competitive edge to their offspring as they commence development ahead of egg-laying species [18, 19]. Oviparous females have been shown to lay approximately 150–180 eggs per oviposition event, with larviparous species producing approximately 50 offspring per event [5, 20, 21]. Some species exhibit both larviparous and oviparous reproduction. [17, 20]. In a study of Calliphora dubia, 70% of females laid only live larvae, 14% laid eggs and larvae concurrently, and 16% laid only eggs, with all eggs nonviable due to the females being too immature [17]. Mackerras [20] also reported that Calliphora augur eggs were produced before females were ready to larviposit and the eggs were consequently not viable. Furthermore, ovoviviparous females have the ability to resorb larvae when no suitable laying site is present [17]. To make matters even more complicated, oviparous females can lay live “precocious” larvae that develop within the oviduct and are consequently many hours older than all other offspring [5, 22].
Female flies must consume protein-rich food soon after their emergence as an adult. This is referred to as anautogeny [5]. Without such provision, their ovaries do not mature to produce fertile eggs but males are fertile regardless of diet [20]. It costs a lot of energy to fly and therefore adults must consume copious amounts of sugary food in the form of nectar, which makes them important pollinators of crops [23, 24].
Larval Development
Larvae digest food externally by secreting enzymes and bacteria that breakdown and liquefy the food source which is then ingested [5]. This is critical given the simplicity of their mouthparts and modification of their digestive system into a straight-forward, tube-like structure. Ammonia is their primary excretory product. Larvae push deep into flesh, necrotic tissue or other substrate while their back ends remain exposed. This allows them to breathe through the posterior spiracles while feeding in liquid anoxic substrates. For larvae to be able to grow, they need to periodically shed their cuticle, which does not expand along with the growing larva. Food sources harbouring bacteria and yeasts will support larval growth more effectively than sterile substrate [25], but it is also well-established that larvae produce antimicrobial substances that inhibit growth of microbes. It is thus likely that suppression of bacterial growth may be selective against particular species, regulating the overall density of microbes.
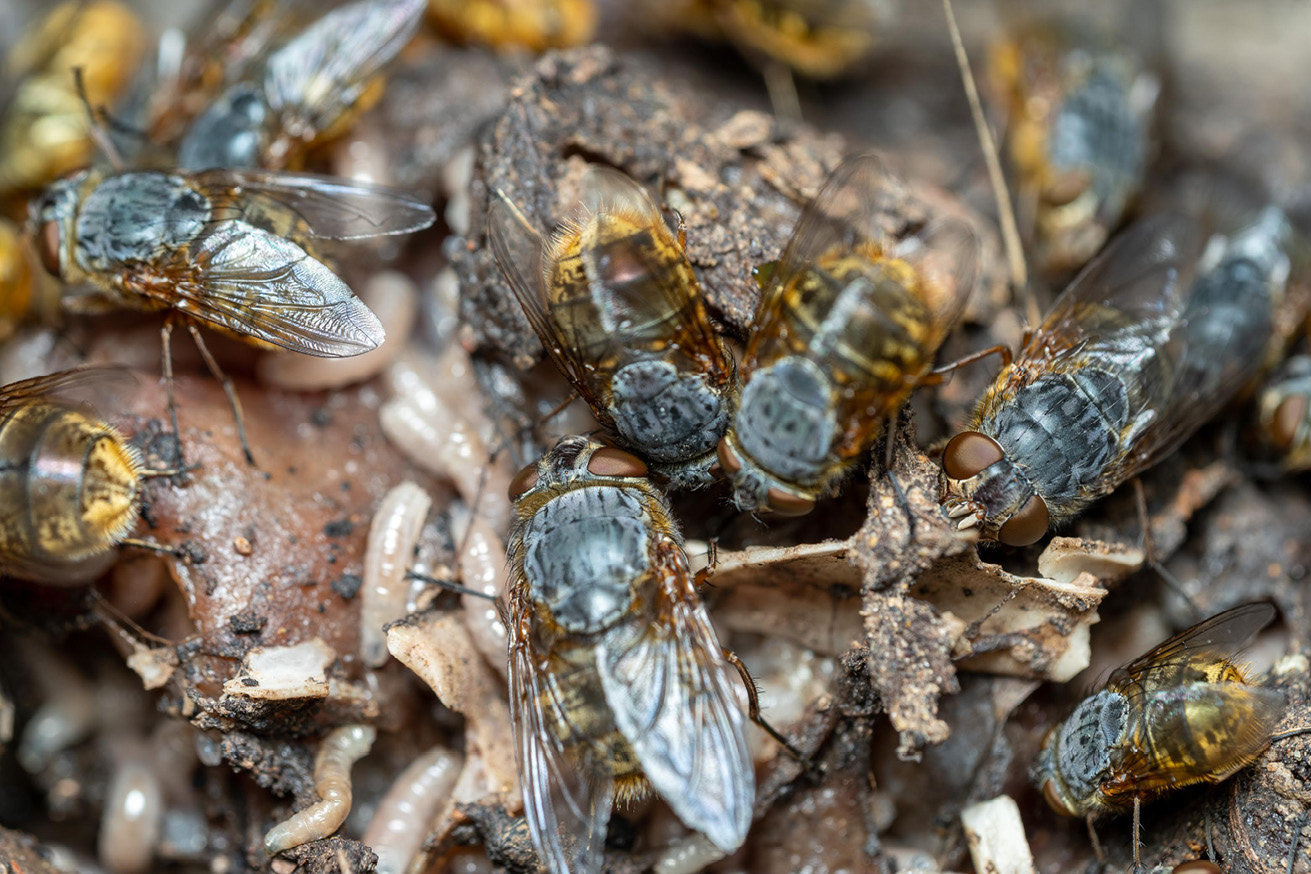
Figure 7.3 Calliphora stygia adult flies and their larvae (maggots) feeding on carrion. Photo by Ash Powell CC BY-NC-ND.
Pupal Metamorphosis
Larvae empty their gut at the cessation of feeding in the third instar [5], and this most likely occurs by digestion of consumed substrate which further adds to energy stores that have been accumulated for the long-lasting quiescent pupal phase that follows. Larvae burrow into soil or under objects where they develop the hardened cuticle (puparium) that protects the metamorphosing insect within (pupa). Pupae breathe through respiratory horns on the fifth segment of the puparium. During metamorphosis, complete remodelling of internal systems occurs, as well as development of external appendages and the segmented body plan (tagmosis). The adult fly emerges using the expansion and contraction of the ptilinum between the eyes, a haemolymph-filled sac that pulsates to break the operculum, a lid-like structure, from the anterior end of the puparium [5]. Over an approximately 24-hour period the newly eclosed fly unfurls its wings, develops pigmentation, and prepares for flight.
Environmental Factors Affecting Calliphorid Fly Behaviour
Because flies are poikilothermic organisms (having varying body temperatures), the activity level, oviposition behaviour, and the developmental rate of immatures are fundamentally dependent on ambient temperatures. Thus, development of immatures proceeds when the ambient temperature is within species-specific temperature thresholds. For L. sericata larvae the optimal temperature for development is 33°C. [20], which corresponds closely to the surface temperature of a human or livestock wound which they frequently colonise. It is common for calliphorid fly larvae to form large aggregations counting many thousands of individuals. This massing generates heat and increases the ambient temperatures that larvae experience, which are sometimes upwards of 50°C. Larvae survive exposure to such potentially lethal temperatures by cycling from the centre to the periphery of aggregations [5, 26]. The exothermic nature of larval masses is a critical issue when estimating the age of larvae in forensic investigations, and when predicting the growth of individuals in research or clinical studies, or laboratory-rearing contexts. Pupal development is also temperature dependent, but the effect of aggregations is not relevant in this stage.
The lower temperature threshold for development is of considerable relevance, because below this temperature, development largely ceases. Adult flies of some species are known to overwinter with the ability to become active on warm winter days—the cold-adapted Calliphora vicina and Calliphora stygia are two examples [20]. Immature flies may suspend their development under unfavourable climatic conditions (diapause) either as larvae, pre-pupae, or pupae [20, 21], only to resume development when triggered by changes in daylength and/or temperature that promise more favourable conditions. Larvae have been observed to successfully feed at both high and low temperature extremes, but may not pupate successfully [27].
Furthermore, ambient temperature affects oviposition behaviour, thus affecting the “colonisation interval” for a suitable substrate. Suitable food sources for colonisation are highly ephemeral, necessitating rapid colonisation by blowflies. This may be highly variable and dependent on humidity, rainfall, wind, light, sun exposure and, fundamentally, temperature [5, 28]. Adults are generally active during daylight hours, although warm evenings have been reported to promote adult activity [29, 30]. When days are mild then flies tend to show a unimodal activity pattern, getting more active as the morning progresses and temperature rises and slowing down again in the afternoon hours when it gets cooler. When days are very hot, a bimodal activity pattern is not uncommon with flies being most active in the morning and afternoon [5]. Having said that, optimal temperatures for adult flight are highly species-dependent and generally dictate species distributions and population density in a given location [31], but the optimal air temperature range for species colonising decomposing remains is considered to lie between 10 and 30°C [5, 29, 31]. For the cold-tolerant Calliphora vicina, egg-laying was shown to occur between 10 and 35°C, while the more temperate L. sericata was shown to lay eggs between 16 and 40°C [32]. Furthermore, adult flight and oviposition behaviours appear to be innately regulated in many species by circadian rhythms. Environmental and climatic conditions aside, reproduction in flies is fundamentally determined by the location and availability of suitable food for the development of offspring. Through the ages, human activity has supplied flies with plentiful organic waste to breed in. For calliphorid flies this has developed into a close (synanthropic) relationship with humans and their settlements.
Calliphorid Interactions with Humans and Domesticated Animals
The requirement for high protein substrate for larval development makes the association of calliphorids with humans and associated animals a natural interaction. Attracted to rubbish and decomposing matter, blowflies have naturally become nuisances when they occur in high abundance. Well-adapted to life associated with humans and our domesticated animals, blowflies are easily spread through human transport within vehicles [5] which helps in the expansion of their natural ranges.
Since calliphorid species will readily feed on a range of proteinaceous, microbially-rich food sources, they are easily maintained taxa for laboratory-rearing, which facilitates research into their biology. A large amount of this research has been in the field of forensic entomology. It has been established that the arrival of species is predictable and can be correlated to particular decomposition stages. This information can be combined with previously established developmental characteristics of the fly species to calculate the postmortem interval, which is the time that has passed since death occurred. Forensic investigators use ambient temperatures to calculate how long it would have taken for the blowfly species that were found on or near the cadaver to develop to the stage at which they were collected. This, combined with the particular colonisation speed for each species, permits fairly accurate determination of the time a person died. In this way, humans have exploited the attraction of flies to protein-rich and microbially-dense environments to provide information in legal investigations.
While often recognised for their role as important nutrient recyclers of dead matter, blowfly larvae may also colonise living organisms (myiasis). Living tissues may be infested by larvae which may be painful and result in the death of the host [5]. Among the cyclorrhaphan flies there are both facultative and obligate parasites of animals. Facultative parasites are species that develop in carrion but will occasionally become parasites in living tissue, while obligate parasites must inhabit a living animal to successfully develop. Obligate parasites include species such as Chrysomya bezziana, the Old World screwworm fly, which requires living flesh for larval development. Most blowfly species are facultative parasites, recognised for their role in forensic science as colonisers of human carrion. The ability to exploit living tissue when it is accessible is a highly successful adaptation [19]. Levot et al. (1979) cite rapid growth through short feeding periods, effective digestive enzymes, few larval instars, and the early colonisation of lucrative decomposing remains as reasons for the immense success of cyclorrhaphan larvae, and the calliphorids are no exception.
Key species recognised as inhabiting carrion include members of the genera Phormia, Protophormia, Cordylobia, Calliphora, Lucilia, Chrysomya and Cochliomyia, and many of these species are also recognised for their role as facultative parasites of living animals. This easy shift likely reflects the microbially rich nature of the food source in both situations. Mackerras & Mackerras [33] indicate that carrion is more attractive to facultative parasites than the flesh of living animals.
Primary colonising larvae secrete proteolytic enzymes including collagenases that externally digest the cutaneous tissue through liquefaction, and larvae gradually invade the subcutaneous tissues, causing shock and septicaemia in hosts [34]. Although L. sericata and L. cuprina are most often responsible for flystrike (myiasis in sheep and other domestic animals), Chrysomya rufifacies, C. stygia, and C. augur were all recorded to also cause myiasis in sheep [20]. In one study, Calliphora augur and sister species C. dubia were recorded to be present in 15% of single species strikes on sheep and in 35% of 1088 strikes in total [35].
Lucilia—Friend or Foe?
Lucilia sericata and L. cuprina are sister species with interesting behavioural inconsistencies, as well as geographically-based differences that make them of significant interest to entomologists. Lucilia cuprina was thought to have originated in the Oriental or Afrotropical regions, while L. sericata was endemic to the Palearctic [36], but they have spread considerably with movement of humans and livestock. They are now both cosmopolitan blowfly species and both are recognised for their role in the colonisation of carrion, although L. sericata more so than L. cuprina. However, this affinity to carrion does not prevent these species from switching to living animals when the opportunity arises.
In Australia, L. sericata is mainly an urban species attracted to refuse. It has limited relevance with regard to flystrike, and was considered by Waterhouse and Paramonov [37] to be “economically unimportant”. Their experiments showed that L. sericata displayed limited oviposition on sheep as compared to L. cuprina [37]. However, in Britain, L. sericata readily laid eggs on live sheep in field experiments [38], and is recognised as the main myiasis-causing blowfly species in sheep in the United Kingdom. Lucilia cuprina is known as the Australian Sheep Blowfly, and is attracted to livestock and generally found in rural areas [37], where it is the primary agent of flystrike. Females lay eggs in the soiled fleece of the animals [5] and larvae feed on the animals’ flesh. Affected livestock may suffer from a large burden of larvae and initial infestation by L. cuprina is often followed by secondary blowfly species. Severe infestations may result in the animal dying and the economic loss to Australian farmers from fly strike is estimated to be in the vicinity of $280 million per annum [39].
Interestingly, L. cuprina females are readily attracted to sheep with clean fleece, but will not lay unless the fleece is soiled with faeces or urine [5]. Fleece-rot results from bacterial infections of Pseudomonas aeruginosa proliferation in moist fleece, causing a green colouration and subsequently becoming highly attractive to female L. cuprina [40, 41]. Thus, it is the bacterial presence that attracts female flies to the area, as seen with the attraction of L. sericata to swarming P. mirabilis on carrion [16]
While myiasis is well-documented to be of detriment in the case of flystrike, there is also a beneficial application of myiasis. Maggot therapy involves the deliberate application of larvae to non-healing human wounds in an effort to debride necrotic tissue, flush the wound with antimicrobial compounds, and stimulate the growth of new tissue [42–44]. Lucilia sericata is commonly the species utilised for this purpose, but L. cuprina has been applied both accidentally [45] and deliberately [46], yielding similar results to its sister species. Thus, the interchangeability of the two species is again established.
Genetic studies have supported the separate species status of both L. cuprina and L. sericata, in spite of strong similarities in morphology and ecology [47]. Hawaiian flies morphologically identified as L. cuprina, however, have been shown to possess L. sericata-type mitochondrial DNA sequences across the COI and COII regions of mitochondrial DNA, which are maternally inherited [48]. According to Stevens and Wall [48] this may suggest a hybridisation event that has become fixed in L. cuprina within Hawaii by lineage sorting. Although hybrids of the two species have been produced in the laboratory but were not highly fertile [37], there have been no reports of true hybrids in the field.
There are two subspecies of L. cuprina. Lucilia cuprina dorsalis is the subspecies dominant throughout sub-Saharan Africa and the Australasian regions [37, 49]. The second subspecies, L. cuprina cuprina has been shown to readily interbreed with L. c. dorsalis in the wild, indicating that despite their varying morphology and behaviour, they taxonomically comprise a single species [49]. This said, varying behaviour and biology between the subspecies may be highly important in the consideration of use of L. cuprina in maggot therapy, but this has not been considered yet given the focus on L. sericata. Future studies investigating the use of L. cuprina in maggot therapy will need to consider possible differences in efficacy and risk.
Summary
The Diptera is a highly diverse insect order but it is the family Calliphoridae (blowflies) that has been the focus of this chapter because many calliphorid species are of great medical and veterinary importance. Calliphorid flies utilise a variety of food sources both as adults and during larval development. Adult blowflies seek the nectar and pollen of flowering plants and are consequently important pollinators. They also visit dung, carrion, and living animals to feed. This means they can potentially spread germs. Generally, calliphorid flies prefer to breed in dead and decomposing organic matter, including carrion, but living animals are also utilised as a food source for larvae. Myiasis, the colonisation of living animals and humans by fly larvae, may be the only way a species of blowfly reproduces, but other species are more flexible and able to utilise both carrion and living animals depending on availability and resource competition.
While blowflies are a nuisance to people and responsible for disease in humans and livestock, many species are also of significant benefit to people beyond their widely-recognised ecosystem services (decomposition, pollination, and food for other animals). Some species that colonise wounds such as L. sericata have a benign and even beneficial impact on wound healing. It is this therapeutic benefit that has led people for thousands of years to use fly larvae (maggots) for the treatment of infected and chronic wounds. Chapters 3 to 6 provide guidance on clinical aspects of maggot therapy and its integration into the healthcare setting [50–53]. In forensic science, it is the knowledge of female colonisation and oviposition habits and species developmental rates that allows law-enforcement to estimate the time of death in coronial or murder inquests.
References
1. Gullan, P. and P. Cranston, The Insects: An Outline of Entomology. 2014: Jihn Wiley & Sons, Incorporated.
2. Thyssen, P.J. and F.S. Masiero, Bioprospecting and Testing of New Fly Species for Maggot Therapy, in A Complete Guide to Maggot Therapy: Clinical Practice, Therapeutic Principles, Production, Distribution, and Ethics, F. Stadler (ed.). 2022, Cambridge: Open Book Publishers, pp. 195–234, https://doi.org/10.11647/OBP.0300.11.
3. Grantham-Hill, C., Preliminary Note on the Treatment of Infected Wounds with the Larva of Wohlfahrtia Nuba. Transactions of the Royal Society of Tropical Medicine and Hygiene, 1933. 27: pp. 93–98.
4. Wu, J.C., et al., Maggot Therapy for Repairing Serious Infective Wound in a Severely Burned Patient. Chinese Journal of Traumatology, 2012. 15(2): pp. 124–125, https://linkinghub.elsevier.com/retrieve/pii/S1008-1275(15)30283-2.
5. Erzinclioglu, Z., Blow Flies. 1996, Slough: The Richmond Publishing Co Ltd.
6. Colless, D. and D. McAlpine, 39: Diptera (Flies), in The Insects of Australia: A Textbook for Students and Research Workers, C.S.a.I.R.O. Division of Entomology (ed.). 1991, Melbourne University Press: Australia, pp. 717–786.
7. Cumming, J.M., et al., Manual of Central American Diptera. 2009, Ottawa: National Research Council Press.
8. Bertone, M.A., Manual of Afrotropical Diptera, Volume 1: Introductory Chapters and Keys to Diptera Families. American Entomologist, 2019. 65(1): pp. 69–70, https://doi.org/10.1093/ae/tmz011.
9. Species2000. Calliphoridae. 2020. https://www.catalogueoflife.org/data/taxon/7KK.
10. Zumpt, F., Myiasis in Man and Animals in the Old World: A Textbook for Physicians, Veterinarians and Zoologists. 1965, London: Butterworths.
11. Wallman, J.F., A Key to the Adults of Species of Blowflies in Southern Australia Known or Suspected to Breed in Carrion. Medical and Veterinary Entomology, 2001. 15(4): pp. 433–437, https://doi.org/10.1046/j.0269-283x.2001.00331.x.
12. Eisemann, C.H. and M.J. Rice, The Origin of Sheep Blowfly, Lucilia cuprina (Wiedemann) (Diptera: Calliphoridae), Attractants in Media Infested with Larvae. Bulletin of Entomological Research, 1987. 77: pp. 287–294, https://dx.doi.org/10.1017/S0007485300011767.
13. Braack, L.E. and P.F. Retief, Dispersal, Density and Habitat Preference of the Blow-flies Chrysomyia Albiceps (Wd.) and Chrysomyia Marginalis (Wd.) (Diptera: Calliphoridae). Onderstepoort Journal of Veterinary Research, 1986. 53(1): pp. 13–18.
14. Barton Browne, L., R.J. Bartell, and H.H. Shorey, Pheromone-mediated Behaviour Leading to Group Oviposition in the Blowfly, Lucilia cuprina. Journal of Insect Physiology, 1969. 15: pp. 1003–1014.
15. Brockelman, W.Y., Competition, the Fitness of Offspring, and Optimal Clutch Size. American Naturalist, 1975. 109: pp. 677–699.
16. Ma, Q., et al., Proteus Mirabilis Interkingdom Swarming Signals Attract Blow Flies. The ISME Journal, 2012. 6(7): pp. 1356–1366, https://dx.doi.org/10.1038/ismej.2011.210.
17. Cook, D.F. and I.R. Dadour, Larviposition in the Ovoviviparous Blowfly Calliphora Dubia. Medical and Veterinary Entomology, 2011. 25: pp. 53–57, https://doi.org/10.1111/j.1365-2915.2010.00894.x.
18. Norris, K.R., The Ecology of Sheep Blowflies in Australia, in Monographiae Biologicae: Biogeography and Ecology in Australia, A. Keast, R.L. Crocker, and C.S. Christian (eds). 1959, Junk: The Hague, pp. 514–544.
19. Levot, G.W., K.R. Brown, and E. Shipp, Larval Growth of Some Calliphorid and Sarcophagid Diptera. Bulletin of Entomological Research, 1979. 69: pp. 469–475, https://dx.doi.org/10.1017/S0007485300018976.
20. Mackerras, M.J., Observations on the Life-histories, Nutritional Requirements and Fecundity of Blowflies. Bulletin of Entomological Research, 1933. 24: pp. 353–362, https://dx.doi.org/10.1017/S0007485300031680.
21. Callinan, A.P.L., Aspects of the Ecology of Calliphora Augur (Fabricius) (Diptera: Callaiphoridae), a Native Australian Blowfly. Australian Journal of Zoology, 1980. 28: pp. 679–684, https://dx.doi.org/10.1071/ZO9800679.
22. Davies, K. and M. Harvey, Precocious Egg Development in the Blowfly Calliphora Vicina: Implications for Developmental Studies and Post-mortem Interval Estimation. Medical and Veterinary Entomology, 2012. 26(3): pp. 300–306, https://doi.org/10.1111/j.1365-2915.2011.01004.x.
23. Cook, D.F., et al., Yield of Southern Highbush Blueberry (Vaccinium Corymbosum) Using the Fly Calliphora Albifrontalis (Diptera: Calliphoridae) as a Pollinator. Austral Entomology, 2020. 59(2): pp. 345–352, https://doi.org/10.1111/aen.12455.
24. Cook, D.F., et al., The Role of Flies as Pollinators of Horticultural Crops: An Australian Case Study with Worldwide Relevance. Insects, 2020. 11(6): p. 341, https://doi.org/10.3390/insects11060341.
25. Hobson, R.P., Studies on the Nutrition of the Blow-fly Larvae . III. The Liquefaction of Muscle. Journal of Experimental Biology, 1932. 9: pp. 359–365.
26. Johnson, A.P. and J.F. Wallman, Infrared Imaging as a Non-invasive Tool for Documenting Maggot Mass Temperatures. Australian Journal of Forensic Sciences, 2014. 46(1): pp. 73–79, https://doi.org/10.1080/00450618.2013.793740.
27. O’Flynn, M.A., The Succession and Rate of Development of Blowflies in Carrion in Southern Queensland and the Application of these Data to Forensic Entomology. Journal of the Australian Entomological Society, 1983. 22: pp. 137–148, https://dx.doi.org/10.1111/j.1440-6055.1983.tb01860.x.
28. Dickson, G.C., et al., Marine Bacterial Succession as a Potential Indicator of Postmortem Submersion Interval. Forensic Science International, 2011. 209(1–3): pp. 1–10, https://doi.org/10.1016/j.forsciint.2010.10.016.
29. Amendt, J., R. Zehner, and F. Reckel, The Nocturnal Oviposition Behaviour of Blow Flies (Diptera: Calliphoridae) in Central Europe and Its Forensic Implications. Forensic Science INternational, 2008. 175: pp. 61–84, https://doi.org/10.1016/j.forsciint.2007.05.010.
30. George, K.A., M.S. Archer, and T. Toop, Nocturnal Colonisation Behaviour of Blowflies (Diptera: Calliphoridae) in Southeastern Australia. Journal of Forensic Science, 2013. 58: pp. S112-S116, https://doi.org/10.1111/j.1556-4029.2012.02277.x.
31. Brundage, A., S. Bros, and J. Honda, Seasonal and Habitat Abundance and Distribution of some Forensically Important Blowflies (Diptera: Calliphoridae) in Central California. Forensic Science International, 2011. 212(1–3): pp. 115–120, https://dx.doi.org/10.1016/j.forsciint.2011.05.023.
32. Ody, H., M.T. Bulling, and K.M. Barnes, Effects of Environmental Temperature on Oviposition Behaviour in Three Blow Fly Species of Forensic Importance. Forensic Science International, 2017. 275: pp. 138–143, https://dx.doi.org/10.1016/j.forsciint.2017.03.001.
33. Mackerras, I.M. and M.J. Mackerras, Sheep Blowfly Investigations. The Attractiveness of Sheep for Lucilia cuprina. Bulletin of the Council for Scientific and Industrial Research, 1944. 181: pp. 1–44.
34. Mauldin, E.A. and J.P. Peters-Kennedy, Chapter 6 — Integumentary System, in Jubb, Kennedy and Palmer’s Pathology of Domestic Animals: Volume 1, M.G. Maxie (ed.). 2016, Saunders Ltd, pp. 509–736.
35. Mackerras, I.M. and M.E. Fuller, A Survey of the Australian Sheep Blowflies. Journal of the Council of Scientific and Industrial Research in Australia, 1937. 10: pp. 261–270.
36. Aubertin, D., Revision of the Genus Lucilia R.-D. (Diptera, Calliphoridae). Linnean Society Journal of Zoology, 1933. 38: pp. 389–463.
37. Waterhouse, D.F. and S.J. Paramonov, The Status of the Two Species of Lucilia (Diptera: Calliphoridae) Attacking Sheep in Australia. Australian Journal of Scientific Research, 1950. 3: pp. 310–336, http://dx.doi.org/10.1071/BI9500310.
38. Cragg, J.B., The Reactions of Lucilia sericata (Mg.) to Various Substances Placed on Sheep. Parasitology, 1950. 40: pp. 179–186, https://doi.org/10.1017/s0031182000018011.
39. Department of Primary Industries and Regional Development, G.o.W.A. Managing Flystrike in Sheep. 2017. https://www.agric.wa.gov.au/livestock-parasites/managing-flystrike-sheep.
40. Emmens, R.L. and M.D. Murray, The Role of Bacterial Odours in Oviposition by Lucilia cuprina (Wiedemann) (Diptera: Calliphoridae), the Australian Sheep Blowfly. Bulletin of Entomological Research, 1982. 72: pp. 367–375, https://dx.doi.org/10.1017/S0007485300013547.
41. Emmens, R.L. and M.D. Murray, Bacterial Odours as Oviosition Stimulants for Lucilia cuprina (Wiedemann) (Diptera: Calliphoridae), the Australian Sheep Blowfly. Bulletin of Entomological Research, 1983. 73: pp. 411–415, https://dx.doi.org/10.1017/S0007485300009019.
42. Nigam, Y. and M.R. Wilson, Maggot Debridement, in A Complete Guide to Maggot Therapy: Clinical Practice, Therapeutic Principles, Production, Distribution, and Ethics, F. Stadler (ed.). 2022, Cambridge: Open Book Publishers, pp. 143–152, https://doi.org/10.11647/OBP.0300.08.
43. Nigam, Y. and M.R. Wilson, The Antimicrobial Activity of Medicinal Maggots, in A Complete Guide to Maggot Therapy: Clinical Practice, Therapeutic Principles, Production, Distribution, and Ethics, F. Stadler (ed.). 2022, Cambridge: Open Book Publishers, pp. 153–174, https://doi.org/10.11647/OBP.0300.09.
44. Nigam, Y. and M.R. Wilson, Maggot-assisted Wound Healing, in A Complete Guide to Maggot Therapy: Clinical Practice, Therapeutic Principles, Production, Distribution, and Ethics, F. Stadler (ed.). 2022, Cambridge: Open Book Publishers, pp. 175–194, https://doi.org/10.11647/OBP.0300.10.
45. Tantawi, T.I., K.A. Williams, and M.H. Villet, An Accidental but Safe and Effective Use of Lucilia Cuprina (Diptera: Calliphoridae) in Maggot Debridement Therapy in Alexandria, Egypt. Journal of Medical Entomology, 2010. 47(3): pp. 491–494, https://doi.org/10.1093/jmedent/47.3.491.
46. Paul, A.G., et al., Maggot Debridement Therapy With Lucilia Cuprina: A Comparison With Conventional Debridement in Diabetic Foot Ulcers. International Wound Journal, 2009. 6(1): pp. 39–46, https://doi.org/10.1111/j.1742-481x.2008.00564.x.
47. Stevens, J. and R. Wall, Genetic Variation in Populations of the Blowflies Lucilia cuprina and Lucilia sericata: Random Amplified Polymorphic DNA Analysis and Mitochondrial DNA Sequences. Biochemical Systematics and Ecology, 1997. 25: pp. 81–97, https://doi.org/10.1017/S0007485300033058.
48. Stevens, J.R., R. Wall, and J.D. Wells, Paraphyly in Hawaiian Hybrid Blowfly Populations and the Evolutionary History of Anthropophilic Species. Insect Molecular Biology, 2002. 11(2): pp. 141–148, https://dx.doi.org/10.1046/j.1365-2583.2002.00318.x.
49. Norris, K.R., Evidence for the Multiple Exotic Origin of Australian Populations of the Sheep Blowfly, Lucilia-Cuprina (Wiedemann) (Diptera, Calliphoridae). Australian Journal of Zoology, 1990. 38(6): pp. 635–648, https://dx.doi.org/10.1071/ZO9900635.
50. Bullen, B. and P. Chadwick, Clinical integration of Maggot Therapy, in A Complete Guide to Maggot Therapy: Clinical Practice, Therapeutic Principles, Production, Distribution, and Ethics, F. Stadler (ed.). 2022, Cambridge: Open Book Publishers, pp. 97–118, https://doi.org/10.11647/OBP.0300.06.
51. Sherman, R., Indications, Contraindications, Interactions, and Side-effects of Maggot Therapy, in A Complete Guide to Maggot Therapy: Clinical Practice, Therapeutic Principles, Production, Distribution, and Ethics, F. Stadler (ed.). 2022, Cambridge: Open Book Publishers, pp. 63–78, https://doi.org/10.11647/OBP.0300.04.
52. Sherman, R., Medicinal Maggot Application and Maggot Therapy Dressing Technology, in A Complete Guide to Maggot Therapy: Clinical Practice, Therapeutic Principles, Production, Distribution, and Ethics, F. Stadler (ed.). 2022, Cambridge: Open Book Publishers, pp. 79–96, https://doi.org/10.11647/OBP.0300.05.
53. Sherman, R. and F. Stadler, Wound Aetiologies, Patient Characteristics, and Healthcare Settings Amenable to Maggot Therapy, in A Complete Guide to Maggot Therapy: Clinical Practice, Therapeutic Principles, Production, Distribution, and Ethics, F. Stadler (ed.). 2022, Cambridge: Open Book Publishers, pp. 39–62, https://doi.org/10.11647/OBP.0300.03.