15. A Fate Worse Than Warming? Stratospheric Aerosol Injection and Catastrophic Risk
© 2024 Aaron Tang and Luke Kemp, CC BY-NC 4.0 https://doi.org/10.11647/OBP.0360.15
Highlights:
- This chapter considers the potential impact on Global Catastrophic Risk of injecting particles into the atmosphere to reflect sunlight, stratospheric aerosol injection (SAI). This both represents a potential technological solution to the threat of climate change and a contributor to GCR in its own right.
- Analyses of potential high impact outcomes from SAI are lacking in contemporary research. This chapter helps resolve this gap by investigating four aspects of SAI’s potential contributions to catastrophic risk: 1) acting as a direct catastrophic risk (through ecological blowback); 2) interacting with other globally catastrophic hazards like nuclear war; 3) exacerbating other risks that cascade and amplify across different systems; and 4) acting as a latent risk that is dormant but can later be triggered.
- It finds that: the potential for major unforeseen environmental consequences seems highly unlikely but is ultimately unknown; SAI plausibly interacts with other catastrophic calamities, most notably nuclear war or an extreme space weather event; SAI could contribute to systemic risk by introducing stressors into critical systems such as agriculture but this is highly understudied; and SAI deployment more tightly couples different ecological, economic, and political systems, creating a precarious condition of latent risk that is the largest cause for concern.
- Across all these dimensions, the specific SAI deployment and associated governance, is critical. A well-coordinated use of a small amount of SAI could incur negligible risks, but this is an optimistic scenario. Conversely, larger use of SAI in an uncoordinated manner poses many potential dangers. We cannot equivocally determine whether SAI will be worse than warming. For now, a heavy reliance on SAI seems an imprudent policy response.
This chapter provides a detailed case study of how and why interventions into climatic change require rigorous analysis. Without investigation into the possible harms precipitated by technological intervention, there is a very real risk that unforeseen consequences could be dramatic. The lessons drawn from this case study are likely instructive for other areas of GCR research, and other examples of generalisable case study research in the field can be found in Chapters 13 and 16.
1. Introduction: Hothouse Earth or Shithouse Earth?
Could the risks of large-scale solar geoengineering be worse than the dangers posed by climate change? Many concerns have been expressed over geoengineering the Earth’s climate. These tend to centre on solar radiation management (SRM) methods, particularly stratospheric aerosol injection (SAI). These range from fears over negative, unintended effects on ecology, political conflict, mitigation deterrence to ethical objections. Given the breadth of objections, it is quite clear that SAI would be iatrogenic in some way. Like some medical interventions, SAI may have adverse side-effects and complications. The question is whether it could be worse than the problem it is seeking to remedy: climate change.
There is a wealth of information on the different risks posed by climate change (although notably little on high-end warming scenarios), yet few attempts to compare this to the potential damages of SAI. This is unsurprising since there have been limited attempts to systematically analyse the myriad of threats posed by SAI.
We address this gap by analysing the severe downside risks of SAI. We do not directly compare the risks posed by SAI and climate change in this chapter. Rather, we provide an analytical foundation for future comparative analyses. In this article we ask: what are the plausible contributions of SAI to Global Catastrophic Risk (GCR)? To the best of our knowledge this is the first attempt to offer a novel, comprehensive framework for comprehending the contributions of SAI to GCR. As noted in Section 2, this is a useful and original step forward for the nascent field of studying GCRs. This is not just simply adding up SAI’s potential negative impacts. It requires understanding how SAI could trigger or worsen other large-scale threats (such as nuclear warfare) or systemic risks. Understanding extreme downside risks can also help provide direction for policy and governance. The future may be hazy, yet avoiding the extreme downsides is a priority for risk management under uncertainty. To guide our investigation, we put forward a novel framework for understanding how SAI, or any other complex risk, contributes to GCR. We then use this to review and discuss the existing evidence on SAI’s critical threats.
In Table 1 we provide a brief set of definitions of the key terms we use throughout this chapter.
Table 1: Definitions.
Term |
Definition |
Climate Engineering |
Large-scale, deliberate interventions into the Earth system to mitigate the effects of negative impacts of climate change.1 |
Extinction Risk |
|
Global Catastrophic Risk (GCR) |
A risk that could plausibly cause a loss in global population of 10–25%2 and a disruption to one or more global critical systems. |
Solar Radiation Management |
Measures which impact the albedo of the Earth system in order to mitigate the impacts of climate change. |
Stratospheric Aerosol Injection |
The injection of light-reflecting chemical, such as sulphur dioxide, into the stratosphere. |
Systemic Risk |
The ability for an individual disruption or failure to cascade into system-wide and cross-system failures3 due to structural conditions. |
Latent Risk |
Risk that is dormant under one set of conditions but becomes active under another set of conditions. |
Termination Shock |
A large and rapid increase in warming after the cessation of SRM measures. |
Buffering |
A period of roughly several months following the cessation of SAI where effects of termination shock do not occur. Redeployment of SAI during this period would ensure that termination shock does not occur.4 |
Our approach makes use of a structured literature review and systems mapping exercise. We use our novel framework to structure a literature review covering studies relevant to the risks of SAI. For each area we highlight the level of evidence and uncertainty, and draw out some key implications. The nature of the risk will depend on the specifics of the geopolitical situation and the SAI intervention. We explore this through a causal-loop diagram (Figure 1) which plots out the connections between the level of risk, the amount of SAI loading, the level of international coordination and other key variables.
Note that for most of this chapter we address SAI in the abstract. The exact potential damage imposed by SAI would vary the way it is deployed. In Section 7 we discuss how the method of deployment creates different impacts. Throughout the chapter we assume a “default” deployment method of SAI to be the continuous multi-decadal global use of planes with multiple injection locations, guided by a global cooperative endeavour led by states with private sector contributions, with an overall objective to respond to global warming. Deployment “thickness” (how much warming is masked) is a particularly important variable. We flag thickness throughout our analysis. Where we discuss the risks of other potential forms of deployment we directly state so.
We proceed by outlining our framework (Section 2), before examining SAI’s direct catastrophic risks (global ecological impacts; Section 3), SAI’s interaction with other catastrophic hazards (Section 4), SAI’s potential input to systemic risk (Section 5), and finally SAI’s influence on latent risk (Section 6). We then discuss how different methods of deployment could lead to different risks and what the policy implications of our analysis are (Section 7). To avoid the critical downside risks we consider throughout the chapter, SAI governance would have to be near perfect for multiple decades.
A solution that is almost impossibly difficult to implement well, and that plausibly threatens catastrophe if implemented poorly, is not a good solution.
Whether this is preferable to climate change remains to be seen.
2. A Framework for Unravelling Global Catastrophe
There is no agreed framework for understanding the contribution of different phenomena to GCR. Most studies and reports on GCRs rely on analysing a set of large-scale “GCR-level” hazards.5 Usual suspects include anthropogenic risks such as nuclear weapons, climate change, and more speculatively, Artificial General Intelligence,6 biologically engineered pandemics, and natural risks such as super volcanoes and asteroids. While there have been some alternative frameworks for classifying GCRs,7 these have yet to be widely adopted. They are also disconnected from relevant literature on systemic risk. Moreover, while they are helpful in classifying a given hazard, they do not act as aids in understanding how much a given event or system could contribute to overall levels of GCR or extinction risk.
There are several problems with the typical, hazard-centric approach. First, it is unclear how these hazards are decided on. Second, a risk is composed of hazards, vulnerabilities, exposure, and response, not just individual threats.8 Third, the different hazards are treated as disconnected when they frequently have similar institutional drivers. Fourth, it ignores systemic risk, particularly the ability for a set of smaller, diffuse risks to scale to a global and cataclysmic level due to the fragility and interconnectedness of critical systems. Any practical framework needs to consider exposures, vulnerabilities, and drivers as well as their interlinkages.
We put forward a four-stream framework for understanding the contribution of a system or event to GCR. This rests not upon having a particular probability of occurring. Instead, we focus on what is plausible (rather than “merely possible”), consistent with our background knowledge of physical and social systems.9 Understanding risks which are plausible, high-impact, but low- or unknown-probability is critical for robust decision-making under uncertainty.10 For example, making decisions on the “better” worst case is central to the Maximin approach. The framework covers the hazard, vulnerability, and exposure elements of risk. Hazards are directly assessed through the first two streams of the framework, while the focus on systemic risk analyses potential vulnerabilities. Latent risk explores the often-neglected possibility of vulnerabilities that are hidden in the short term. Exposure is articulated throughout the analysis. Response (i.e. SAI governance) is discussed throughout Section 7.1.
Our four-stream model looks at direct contributions to GCR, how it could potentially trigger other high-impact risks, its contribution to systemic risk in global critical systems, and its capacity for latent risk. Across each, we also consider potential feedback loops between SAI and each stream. Our four-stream model is as follows:
- The first stream focuses on directly catastrophic impacts. A direct contribution refers to ways in which the impacts caused by SAI could alone plausibly cause sufficient mortality and morbidity without considering wider social knock-on effects.
- The second stream examines how SAI could interact with other high-impact hazards such as nuclear war.
- The third investigates how SAI could contribute to and be affected by systemic risk. Systemic risk focuses on how structural conditions and multiple small stressors can lead to widespread collapse or synchronous, reinforcing failures.11 Indeed, complex systems can undergo rapid degeneration even without large shocks. They frequently organise into critical states in which small perturbations quickly cascade into calamity.12
- The final stream focuses on SAI’s latent risk. Latent risk focuses on deciphering how SAI could pose threats that manifest under post-catastrophe conditions, such as in the aftermath of societal collapse.
Together, these different factors provide a comprehensive framework for comprehending how SAI could raise or lower overall levels of GCR in the world. The framework is intended to be a first step to risk comparison, in this case climate change and SAI. These streams echo the channels of risk discussed in “Climate Endgame”.13 This helps make any risk-risk comparison easier. While the framework is extensive our application is limited. Due to time and resource constraints we only explore the most well-evidenced and likely risk channels, leaving others relatively untouched, such as the impact of SAI on the likelihood of biologically engineered pandemics.
Historically, comparison between the two has been a rhetorical device to justify SAI. This is by no means a straight-forward juxtaposition since the two interact (for example, through mitigation deterrence: actors may be less open to ambitious emissions reduction if there is a “technofix” on the horizon14) and any analysis hinges on subjective judgements about climate sensitivity, tipping points, adaptive capacity, and the likelihood of international cooperation. There is also the issue of which precise baselines should be used for comparison:15 what should climate change or SAI be specifically compared against? In addition, how should the two be compared? Given the high uncertainties for both climate change and SAI, is a Maximin analysis of the “better” worst case a prudent or viable approach? Given these difficulties, we do not look to provide a definitive answer or quantitative analysis. Ultimately, we are not just comparing two different sets of risks, but two separate Earth system states16 with different winners and losers. Navigating these entangled risk analyses is an area for future analysis, but analysis that this chapter can hopefully inform.
Nonetheless, any public deliberation and democratic decisions need to rest on comparable evidence and information. Any action is bettered by risk assessment, even if it is always mired in uncertainty. This article provides an initial and incomplete basis for informing such discussions. Imperfectly mapping out risk trade-offs is preferable to sleepwalking17 into a dangerous future.
3. Directly Catastrophic Impacts: Ecological Blowback?
Could SAI lead to directly18 catastrophic ecological impacts? Existing studies highlight a raft of potential negative consequences. But the specific nature of these impacts, and their contributions to catastrophic outcomes, depends on the specific SAI implementation. This is an issue of high uncertainty, particularly regionally.
The projected local ecological effects of SAI are mixed and uncertain, depending on the specific analytical approach and specific SAI deployment. Monsoon areas would likely face a drop in precipitation under large scale SRM,19 but this focuses on SRM in the abstract and may not be fully applicable to SAI. Many regions could face a seasonal under- or over-compensation in rainfall (compared to a high warming average (RCP 8.5) from 2010 to 2030, and assuming SAI is implemented to mask five degrees of warming).20 Effects on hydrological systems would be regionally diverse and uncertain due to potential changes in nonlinear variables including surface runoff, evapotranspiration, rainfall levels, and distribution.21 These fine-grained changes in weather could then affect vegetation. Plant communities could transform their structure, traits, and geographical range, particularly under larger swifter SAI deployments.22 While SAI might offer salvation to climate vulnerable vegetation it will depend on deployment timing. Some communities may already be committed to at least local extinctions before SAI is deployed. SAI would likely result in ecological trade-offs with some communities benefitting and others suffering. The exact nature of these trade-offs is uncertain and needs further study.23 The key theme here is that SAI would likely have a range of impacts on many ecological systems. But how these would play out is highly uncertain, particularly at regional scales. Impacts hinge on the inherent uncertainties within complex ecological systems, varied comparative baselines, and the specific SAI deployment.
The overall direct impacts of SAI, while uncertain, do not currently seem to constitute a catastrophic threat. Whether SAI would cause greater risks in terrestrial, freshwater, marine systems than climate change is unclear and depends on SAI’s specific deployment configuration. Higher levels and swifter deployment of SAI would mean greater potential for disastrous impacts.24 Additional considerations like seasonal25 or hemispheric26 deployment further affect potential impacts.
There is a paucity of research on SAI impacts,27 particularly so for catastrophic or worst-case impacts. This has been the case for climate modelling literature in the past as well.28 Climate modelling is often an exercise in “betting on the best case”.29 Others have noted this idealistic tendency for SAI modelling:30 for example, limiting SAI use to only halving warming31 or limiting SAI deployment to spring.32 These idealised approaches in theory could reduce negative impacts associated with SAI. Yet their likelihood is questionable due to optimistic assumptions of multi-decadal international cooperation (see Section 5.2).
The possibility of dangerous ecological tail-risks depends on the level of cooling. Initial game theoretic research indicates the possibility of overcooling if SAI is pursued by uncoordinated actors.33 Negative impacts which are projected to be relatively minor in existing studies — for example, sulphate deposition impacts on terrestrial ecosystems34 — may become major ecological issues if SAI is deployed to far more of an extent than envisioned. Similarly, a poor choice35 of aerosols could result in large-scale ozone depletion.36 It is unclear whether, in these extreme cases, biophysical impacts would revert to their pre-SAI state once SAI is removed. Modelling on “worst” cases is thus critical in informing SAI’s desirability. Exploring uncoordinated scenarios with the (simultaneous) use of different aerosols, different desired extents of cooling, and implementation by a small club, would all be helpful complements to existing idealised modelling scenarios.
Regardless of how developed our understanding on SAI impacts become, there will always be inherent uncertainty. When dealing with a complex system like the climate there is always the chance that a black swan is lurking in the dark.
Some commentators have downplayed the potential of unknown impacts due to the availability of historical analogues, namely historically severe volcanic eruptions.37 Improvements in modelling, a gradual implementation, and a cessation if unacceptable negative impacts are found could also lessen the likelihood of an unforeseen catastrophic tipping point.
None of these reasons are causes for comfort. Modelling, regardless of improvements, may simply be incapable of capturing rare tipping-points and is not intended to accurately predict or foresee non-rational political dynamics.38 In addition, a gradual rational phase-in and phase-out relies on optimal governance conditions. Overly rapid deployment due to “free-driving”39,40 or overly slow phase-out due to technological or infrastructural lock-in41 are entirely plausible. Moreover, SAI impacts may also not follow the pathway of historical analogues. The core rationale of SAI is to manufacture the cooling effect of a volcanic eruption in a “safe” manner, not replicate volcanic processes. Deviance from historical analogues is especially a possibility if the choice or mix of aerosol is radically different. This is particularly the case since climate change and human-pressures are already pushing ecological systems into novel states.42 SAI would push systems into further novel states that make unseen ecological responses likely.43
Our understanding of both Earth systems and the likely contours of deployment are too weak for us to rule out a potentially catastrophic form of ecological blow-back. For now, the literature points to SAI having numerous impacts. But none seem remotely capable of being a GCR, particularly if SAI deployment were limited. Nonetheless, the spectre of an unforeseen tipping point in the Earth’s climatic system remains.
4. Interactions With Other Global Catastrophic Hazards (GCHs)
The impacts of SAI, or any other catastrophic risk, should not be assessed in isolation.44 Different catastrophic hazards45 have interactions. One could potentially trigger another and/or worsen its effects. Climate hazards, for example, have been shown to compromise governments’ ability to provide effective responses to COVID-19.46 The potential for one global shock to ignite and amplify another has previously been dubbed “double-catastrophes”.47 Baum, Maher Jr. and Haqq-Misra (2013) suggest that this could be the case if nuclear war or a pandemic were to disrupt an SAI system, leading to abrupt termination shock. GCHs which are simply a matter of probability, like extreme space weather or a volcanic eruption, may also coincide through pure bad luck.
In this section we consider both a broader array of hazards and how SAI could trigger and interact with them. This will not be an exhaustive comparative analysis of all possible GCHs. Instead, we focus on hazards that have clearly established causal relationships, relatively well-developed literatures, and some empirical track record of their impacts. Our analysis suggests that the possibility of SAI sparking other GCHs are tenuous. SAI could only plausibly contribute to large-scale conflict and potentially nuclear war. The possibilities of SAI exacerbating other GCHs are more concerning. SAI has the worrying ability to significantly heighten the impacts and mortality of any global catastrophe due to termination shock.
4.1 Volcanic eruption
A large volcanic eruption would demand rapid SAI adjustments. While severe overcooling seems unlikely (the cooling of SAI and volcanic winter are not additive),48 SAI should be rapidly scaled down in a matter of weeks.49 Laakso et al. (2016) assume a relatively thick SAI injection (offsetting roughly a doubling of carbon dioxide from preindustrial levels). The prudent course of action for thinner SAI is unclear. However, the SAI adjustment in a volcanic future is not simply one of scale down. SAI injection may need to increase in the opposite hemisphere to the volcanic eruption to ensure a more uniform global temperature50 (a high temperature variance across hemispheres can have severe adverse impacts on precipitation and drought dynamics).
Adjusting the SAI level may seem straight-forward but depends on an informed, rapid political response. There are reasons to doubt this would be forthcoming. First, the technical demands may prove too much for cumbersome domestic and multilateral politics. These include potentially politically vexing dilemmas over the balance between scaling SAI up and down on different hemispheres, whether to inject SAI at new locations or “thicken” existing deployments,51 or whether SAI should be scaled down at all. A second and novel addition is that a volcanic eruption would not solely affect temperature. Many pinch points of global supply systems are near active volcanic areas. Even modest volcanic eruptions could lead to disruption and catastrophic economic system collapse.52 The difficulty of coordinating regional SAI adjustments would be compounded by sub-optimally functioning supply systems and general economic and political chaos.
While the interactions between a volcanic eruption and SAI currently seem to have only modest direct contributions to catastrophic risk, the highly political decisions of a volcanic-SAI world may lead to political ruptures and ineffective SAI governance.
4.2 Space weather
Solar flares, coronal mass ejections, and associated solar radiation and geomagnetic storms, can lead to widespread damage to terrestrial, avionic, and space infrastructure. The fear for SAI is that a “black sky” event could disrupt and knock out critical SAI infrastructure. Yet there have been no attempts thus far to investigate SAI-space weather interactions. We examine SAI interactions with an Earth-bound space weather event roughly on par or worse than the 1859 Carrington Event — the benchmark for extreme space weather events.53 A current-day Carrington Event would likely lead to widespread electrical failure and disruption for multiple months at minimum, potentially years.54
Extreme solar events are difficult to accurately and timely forecast. They are essentially random events55 which provide little forewarning. Solar radiation can travel at such high speeds that an extreme coronal mass ejection would likely reach Earth in less than a day. Other radiation and energised particles travel at or close to lightspeed — eight minutes to reach Earth. Even with the earliest detection possible there would be little response time.56 It would be a late flinch to an oncoming blow.
The impacts of extreme space weather events are vast. Aviation, satellite, and general electronic infrastructure are especially vulnerable. Energised particles can affect memory cells — for example, changing a bit from a 1 to 0 and vice versa — that lead to erroneous commands or overall hardware failure.57 Global navigation and communication systems would experience disruption and downtime that could last several months (alternative navigation systems, like the US Alternate Position Navigation and Time programme, may still be affected by electrical damage).58 Aircraft crew would have greatly limited airtime due to limits of safe radiation exposure.59 Flights at higher altitudes and closer proximity to the Earth’s poles would be unlikely to continue.60 The use of automated aircraft would be compromised by widespread electrical and avionic damage. Especially alarming is that SAI would likely depend on vulnerable aviation, satellite, and general electronic infrastructure for deployment, monitoring, impact attribution determination, calibration, and modulation.
Impacts of space weather events are not limited to human infrastructure. Substantially increased UV output can influence the Northern Hemisphere jet stream, ozone production (and ozone UV absorption and warming), and precipitation patterns.61 These systems, particularly precipitation, are the same systems that SAI is likely to greatly affect. Interaction between these impacts is currently unclear.
These disruptions appear enough to halt even a robust SAI system. Even with high uncertainties of potential infrastructural impacts62 and the nature of the event itself,63 the limited evidence so far indicates that SAI infrastructure would be vulnerable and exposed to damage, thus leading to termination shock if SAI was sufficiently thick (see Section 6). In the aftermath of an extreme space weather event, continued implementation or preservation of SAI infrastructure would have to compete for limited government attention. Damage would be widespread and international — ranging from railway failure64 to power failure65 to failure of satellite infrastructure.66 Governments and resources would be stretched thin and SAI reimplementation may be neglected. An extreme space weather event could lead to severe economic and infrastructural shocks67 that make continued SAI deployment infeasible. At worst, widespread power failures could lead to ripple effects across food, health, and transport systems that extend recovery time potentially into decades, driving modern societies back to a more fractured pre-electronic state.68 It is unclear how SAI, with its high technical and information demands,69 could continue under these conditions. Troublingly, mitigation options are currently limited and highly depend on future (but relatively well-known) scientific and engineering solutions.70 Considering the speed of space weather events, SAI infrastructure would have to be built to be resilient (with technology which does not currently exist) from the offset.
SAI is ultimately highly vulnerable to extreme space weather events. Widespread electrical damage would compromise SAI redeployment, making a termination shock highly likely and worsening the already catastrophic impacts of an extreme space weather event.
4.3 Nuclear weaponry
SAI would likely worsen any nuclear winter and our recovery from it. A nuclear war could occur due to either an accidental strike leading to escalation, or a full-blown exchange. Even a relatively smaller conflict between Pakistan and India would have global ramifications. The background risk of incidental or inadvertent nuclear deployment is present unless there is total nuclear disarmament.71 In addition to nuclear winter, the physical blast, ionising radiation, and electromagnetic pulse (EMP) would all contribute to widespread and severe damage of electronic infrastructure,72 including SAI infrastructure. Indeed, EMPs are similar in effect to the “black-sky” events discussed in Section 4.2. This leads to two key concerns. The first is the combination of SAI cooling with nuclear winter conditions, the second is the grim mixture of nuclear cooling combined with termination shock.
The combination of SAI’s existing cooling and additional nuclear winter would likely lead to short term overcooling, followed by medium- or long-term overheating due to termination shock.73 It could be global frost followed by global furnace. Alternatively, there may be the potential for SAI and nuclear winter layering to spark non-linear or unexpected cooling effects. This is an area that justifies further study. There is modelling on the impacts of a nuclear detonation, comparison of nuclear and climate threats via the “climate-nuclear nexus” (Scheffran et al., 2016), and modelling on the impacts of SAI deployment and termination shock. Yet so far nothing integrates these two separate bodies of knowledge. The oversight is interesting given the entangled histories of climate science and nuclear weapons research.74 For now, the interactions between nuclear winter and SAI remain neglected and our analysis here is hence provisional. In any case, such rapid swings in global temperature would be unprecedented for the Earth system and humanity.
A key question is whether a disrupted SAI system could be revived during nuclear winter to prevent a termination shock summer, and whether SAI was masking sufficient warming for termination shock to occur (see Section 6). But there also are reasons to believe that the re-establishment of an SAI system would not be able to occur during the buffer period in the wake of a nuclear cataclysm. First, technological damage may be so severe that timely deployment is impossible. Backup infrastructure like aircraft (and associated supporting infrastructure such as air traffic control) may be damaged beyond repair or be grounded for security purposes. Second, political and policy attention would likely be focused on other post nuclear issues, such as disaster recovery and the creation of alternative food systems. As with other disasters, governments would be stretched thin and may prioritise these more short-term issues. Lastly, a post-nuclear world would likely exhibit a lack of international cohesion that is seen as an enabling condition for effective SAI.75 Discussions over SAI have already been deadlocked.76 It seems unlikely that a world of post-conflict lessened trust would be more conducive to speedy decision-making. Different countries may drop out of implementation, further complicating SAI deployment configurations, possible regional impacts, and concordant policy responses. Disagreement over resource allocation is likely to arise, as is the case for many disaster recoveries.77
The presence of thick SAI greatly increases the potential consequences of nuclear warfare, and vice versa. The rapid temperature swings involved with a nuclear winter and termination shock summer would likely lead to ecological disaster, and a chaotic post-nuclear world would not likely reimplement SAI in a timely sensible manner.
4.4 Pandemics
A pandemic that reaches the level of a GCR could be enough of an economic or population shock to sever an SAI system.78 Whether the system could be reactivated during the buffer period would depend on both the severity as well as the length of the pandemic. COVID-19 provides a chilling reminder that states are not rational nor necessarily cooperative during a disease disaster. COVID-19, a far cry from being a GCR, has spawned fragmented responses and cases of both vaccine nationalism and vaccine diplomacy. Such multilateral behaviour does not engender confidence that a pandemic with a significantly higher mortality rate would lead to survivors coolly and collectively reactivating an SAI system whilst dealing with the outbreak. Other issues, like keeping healthcare systems afloat, would likely be an overwhelming priority. With resources and capacity stretched thin, SAI may be neglected. A pandemic would be a severe shock to political and economic systems that may preclude continued SAI use, not least rational, well-governed, well-resourced SAI use. Whether this risks termination shock depends on the amount of warming masked.
There are also reasons (albeit speculative) to believe that SAI could contribute to a pandemic. SAI induced temperature changes and uncertain regional climatic effects can alter disease transmissions.79 This could in turn affect pandemic dynamics. As with general ecosystem impacts (Section 3), a larger and quicker SAI deployment can be expected to have more severe impacts. Critical nodes in urban and health systems may become exposed to diseases that are beyond typical immunity or resistance (see Section 5.3 more on SAI-health interactions). This could be the spark for a pandemic spread, particularly if decision-makers are unprepared to make early and rapid response measures. However, the most worrying (but thus far neglected) concern would be effects on animal populations. Similar concerns of low or lapsed immunity or resistance would apply to animal populations and new disease vectors. But animal populations would lack similar healthcare systems to keep disease spread at bay. Many contemporary pandemics have resulted from cross-species spillover,80 including the 2009 Swine Flu Pandemic from pigs and birds, and the 2013–2016 Ebola Epidemic and COVID-19 Pandemic from bats. Altered animal disease dynamics, particularly those stemming from unpredictable regional SAI impacts, may increase the frequency and severity of future pandemics.
5. The Systemic Risks of Climate Engineering
Both previous societal collapses and disasters in the modern world are marked more by the accumulation of many stresses leading to failure, rather than single abrupt shocks destroying systems.81 Seemingly modest stressors can cascade to catastrophe. This section analyses the potential of SAI to create and be impacted by biophysical and political stresses which contribute to global systemic risk.
The world currently exists in a deeply interconnected, and increasingly homogenous state which is prone to systemic risk.82 One ship blocking the Suez Canal in March 2021 led to losses of roughly $6–10 billion.83 More serious stressors could lead to far more severe consequences. The economic and political state of the world would be central in determining whether risk cascades. It is unclear how SAI could or would adjust the structure of the globalised economy. Hence, instead we focus on a few critical systems that SAI might be expected to impact and where there have been initial attempts to gather evidence: agriculture, health, and international politics.84 SAI would likely not alter any of these system structures, but would rather aggravate existing systemic vulnerabilities.
5.1 Agriculture
SAI’s effects on temperature and precipitation distributions would likely affect agricultural systems. The precise nature of these impacts are unclear.85 For example, some studies have shown that the low temperature, high carbon dioxide environment of a SRM deployment might increase yields: maize yields may increase in China,86 as could overall global yields of maize, wheat, and rice.87 On the other hand, solar dimming might reduce yields of groundnut in India88 or offset benefits of reduced temperature.89 These effects would all further differ across crop and area. The differing approaches to analysis (Xia et al. (2014) focus on SRM to offset a 1% increase in carbon dioxide from preindustrial levels for 50 years, whereas Pongratz et al. (2012) focus on SAI masking carbon dioxide concentrations of 800 ppm) as well as use of outdated equatorial injection in these studies (see Section 7.1) make clear conclusions difficult to discern. The main point is that SAI would affect agriculture, but the precise impacts are unknown.
Regardless, the sensitivity of these key staple crops alone is a cause for concern. Small variations in yields of staple crops could induce disproportionate price fluctuations and cascades into socio-political violence, particularly in areas with political instability and weaker governance.90 Additional uncertainties with attribution between SAI and agricultural yields could compound potential political difficulties.
Even in the case that SAI provides agricultural benefits, these are likely to be marginal if other issues affecting agricultural productivity, such as habitat loss and soil degradation, continue unabated.91 An SAI high carbon dioxide, low sunlight world would also require additional adaptation on the part of agricultural actors. This does not look likely given agricultural adaptation to climate change has so far only been modest.92 Large-scale changes in yield and precipitation are likely to create at least short-term food insecurity. There is evidence that existing population density and economic growth are closely tied to the existing climate niche. The narrow climatic envelope of ∼13 °C has provided beneficial environmental conditions within which most humans and societies have tended to historically cluster.93 Our agricultural systems almost certainly are similarly tied to this niche, and any sudden change at a global level is likely to affect short-term yields and prices.
5.2 Politics
SAI could feasibly spark conflict and instability. There are already some emerging empirical links between food price shocks and socio-political violence. Moreover, the very act of undertaking SAI could be grounds for dispute. States may look to develop their own SAI capabilities before others do, creating more extensive backup infrastructure to avoid dependencies on others, or even construct counter-SAI capabilities.94 Existing political order may become undone by SAI.95 A novel and interesting example could be high historical emitters like the US using the Common but Differentiated Responsibilities and Respective Capabilities principle as an instrument to assert SAI control or leadership (“we are mostly responsible for climate change, therefore it is ‘just’ that we lead the response”). Manipulation of the climate could become a new frontier for political conflict or even warfare. Different cross-boundary impacts on different regions would create large sets of winners and losers, alongside questions of attribution96 and compensation. Whether such disputes could snowball into conflict is beyond prediction. Nonetheless, it is reasonable to say that unless enacted as altruistic, cooperative endeavour over multiple decades, the project of SAI would load further pressure onto existing international tensions. But even in the most altruistic cooperative scenarios, there may still be sub-national tensions in and/or between “donor” and “recipient” populations.
There is also the possibility that politics would worsen SAI. SAI and politics is a two-way street. Political conflict can cascade to affect SAI deployment and its impacts. Previous studies have made a compelling case that the direct weaponisation of SAI is unlikely.97 High-impact uncertainties, management difficulty, low precision, and preferable alternative weaponry make SAI an unappealing instrument in state arsenals. However, this does not mean that SAI has limited military use. SAI may not have usefulness as a direct weapon, but can function as a support system or a threat. Indeed, early attempts at cloud seeding were used by the US military during the Vietnam War as a tactical weapon to extend the Monsoon Season and disrupt North Vietnamese supply lines (Operation Popeye).
Another avenue for political dynamics to worsen a SAI deployment, and that has received relatively little attention, is via cyberwarfare. In May 2021, a ransomware cyber-attack forced a US fuel pipeline out of service. A $5 million ransom was paid to restore service.98 As a globally critical (and potentially highly politicised) piece of infrastructure, SAI would likely be a target for private or state actors. SAI deployment dependent on any software or advanced algorithmic system,99which is likely given the high technological and informational demands of deployment,100 would be vulnerable to cyberattack.
Cyberattacks do not need to come from external forces. For instance, the notorious 2000 Maroochy Cyberattack was from a disgruntled ex-employee.101 SAI would likely depend on a large workforce and have numerous reasons for controversy.
These political dynamics would have decades to play out. A cooperative and benevolent deployment of SAI could crumble into chaos with a change in actor preferences (or vice versa). Politics and its broader conditions are likely to change substantially over coming decades. Interactions between future geopolitics, warming and emissions, and technology are all nigh impossible to predict or even foresee,102 but would be of critical importance to SAI and its governance. Relying on one set of optimal political assumptions would be greatly unwise.
5.3 Health
SAI could negatively impact human health by both changing disease vectors and range (and therefore pandemics, see Section 4.4), and by undermining existing health system infrastructure. The regional variations of SAI’s impacts on temperature and other ecological factors would likely affect disease transmissions. SAI-induced reductions in monsoon rainfall may increase cholera risk,103 and temperature changes can affect transmission of vector borne diseases like malaria.104 Yet such health impacts are chronically understudied: currently only four papers focus on the health impacts of SAI.105 The lack of coverage is significant since these studies have critical limitations, namely an assumption of equatorial injection (see Section 7.1). The impacts of other forms of deployment are largely unknown. Similarly, there is little research on the health impacts of exposure to SAI aerosols,106 and the few quantitative assessments of mortality related to air quality and changes in UV exposure carry significant uncertainty.107
Despite these limitations, the research to date does point towards potential dangers. Alterations of disease transmission are especially important because diseases may reach populations which have lapsed or little immunity or resistance,108 or may have relatively weak or vulnerable public health systems. These critical nodes in health and urban systems, which otherwise would be less exposed, may amplify health risks and impacts: an epidemic may be amplified to become a pandemic (Section 4.4). The uncertainty of SAI’s potential deployment configurations, associated impacts, and state of existing health systems means that early identification of different critical nodes would likely be difficult and insufficient. Overall, systemic effects between health and SAI currently seem modest and carry high uncertainty. However, they are not negligible.
6. Latent Risk and SAI
Latent risk refers to risks that are dormant, but could become manifest during times of heightened societal vulnerability. The most obvious example would be the additional risks that arise in the aftermath of a collapse (widespread, significant, and enduring loss of life, political organisation and economic capital) or another global catastrophe, for example violent conflict over food and water. Latent risks are particularly important as they can provide one tangible way in which recovery from global shocks could be undermined and spiral towards extinction risk.109 We have already dealt with these partly in Sections 3–5. In short, latent risk is perhaps the largest risk factor for SAI. SAI changes the nature of climate risk by making the “likely” outcomes less severe, but making “less likely” (or “fat-tail”) outcomes substantially more severe. The risk of termination shock thickens the tail. Large amounts of SAI loading could create a precarious condition in which any sufficiently large global shock is likely to be compounded by a tumultuous termination shock. It is in these worst cases where SAI becomes clearly worse than worst case climate change.
While there is subjectivity as to what a “threshold” for termination shock would be, Parker and Irvine (2018) suggest a SAI cooling threshold of around 0.3 degrees, implying a termination of at least 0.15 degrees warming per decade. Kosugi (2013)110 puts the termination threshold at 0.2 degrees, implying an SAI cooling threshold of around 0.4 degrees.
The speed of termination shock depends on the form of SRM. SAI has a half-life of approximately eight months (approximately half the levels of coolants would still be present after eight months) and warming would still take several years to reach its unmitigated levels.111 Depending on the amount of warming masked, SAI has a distinctly high latent risk due to termination shock. A temperature rise of six degrees in the space of centuries would be an order of magnitude faster than the warming experienced during the Great Permian Dying.112 If experienced in a period of decades, it would be an order of magnitude faster still. Current warming rates are geologically unprecedented; this speed would be chillingly rapid.
Critics have framed termination shock as an overblown problem for numerous reasons. These include that countries are unlikely to willingly reverse SAI, that there would be a sufficient buffer period to resume SAI, and it is unlikely to be hiding a large amount of warming.113 These all seem to align with the inclination for both modelling and analysis of geoengineering to focus on the “best case”: that there would be sufficient cooperative governance and deployment of SAI, that there would be rational responses to any system lapse or shock, and that SAI would be used to only shave-off a small amount of warming.114 Yet, SAI is widely portrayed as an emergency response: it is most likely to be used in a worst-case high warming scenario, not a best-case limited warming one. Moreover, the likelihood of high-end warming, governance fragmentation, or another GCR occurring are all disarmingly large.
The likelihood of a catastrophe curtailing SAI efforts and causing termination shock is usually dismissed as very low. This is likely mistaken. We have covered some of these catastrophes in Section 4. While there is considerable uncertainty, the likelihood of a GCR in the coming centuries does not appear to be vanishing. Estimates for a large-scale space weather event over the next decade or so range from 0.46%115 to 20.3%.116 Estimates of the probability of nuclear war are few and vary, but one model of inadvertent conflict between the US and Russia using historical data put it at 0.9% per year.117 SAI could also be slowly scaled back as mitigation and CDR efforts increase.118 But this would likely require multiple additional decades which would (assuming no mitigation of other global threats) incur a higher likelihood of another catastrophe striking.
A more compelling retort is that SAI could be reintroduced within years at a reasonable cost. Some have suggested that given that SAI could be run at >1% of the GDP of the G20 and hence even losses of 75% of GDP (an unprecedented economic disaster) would be insufficient to keep an SAI system deactivated.119 Such analysis overstates the coherence and rationality of states responding to crisis. The value of an extra three billion doses of COVID-19 vaccines would provide benefits of $17.4 trillion, at a cost of around $18–120 billion.120 Yet vaccine production remains chronically low. Even in far less dire circumstances we can clearly not trust decision-makers to take the optimal course of action.
SAI can be seen as one vast project to make the climate system more tightly coupled and synchronised with the global economic system. From a resilience perspective, such efforts are a liability. It makes it far more likely that the failure of one system will spill over into another, sparking non-linear feedback loops that result in “synchronous failures”.121 There are of course ways to make such complex engineering systems more resilient and robust, namely via backups and redundancies. However, current economic incentives for efficiency (particularly via cost reduction), mean that strong redundancies are rarely in place. SAI redundancies specifically are likely to be expensive and thus inconsistently implemented.122 In any case, it is unclear what redundancies would be effective at making an SAI system catastrophe-proof. Making SAI resilient to natural disasters or terrorist attacks seem relatively straight-forward,123 but the same cannot be said of a true global catastrophe.
The inherent unknowns of highly complex technological systems also contribute to the possibility of termination shock. Highly complex systems, like SAI would be, are prone to “Normal Accidents”.124 Large-scale accidents and disruptions are to be expected in sufficiently complex and tightly coupled systems. Unforeseen technological failures are simply a fact of life.
While latent risk is a genuine concern, it is a danger for only the greatest threats on the horizon and the “thickest” SAI deployments. A true, dramatic, global calamity would be needed to both disable an SAI system masking a large amount of warming and keep countries either preoccupied or incapable of reinstating it for several years. In this context, the risk comparison between SAI and climate change becomes clearer. SAI’s worst case outcomes through severe termination shock are worse than the worst cases of climate change.
In Table 2 we summarise our analysis of direct impacts, GCH interactions, systemic risks, and latent risk.
Table 2: Summary of SAI’s direct impacts, GCH interactions, systemic risks, and latent risk.
7. Discussion: Building the Policy Boundaries for Climate Engineering
7.1 The means of deployment
Our analysis thus far has assumed a “default” deployment of optimal conditions of a global material approach to mitigate climate change. This is not necessarily the most likely scenario and the means of deployment and context will dramatically impact SAI’s catastrophic risk profile. One of the critical variables to consider is the overall objective of a SAI deployment.
There are multiple potential objectives of SAI deployment, ranging from temperature reduction (of different extents), precipitation impact management, to biodiversity conservation.125 These objectives will also depend on existing emissions reduction policies. There are also multiple potential “design” options for deployment configuration,126 ranging from deployment timing, extent, placement, to aerosol selection.127 The extent of cooling for example not only depends on how much aerosol is released, but the height of injection in atmosphere (lower stratosphere injection produces more cooling).128 Much of the existing study on SAI assumes injection along the equator.129 Equatorial injection is the most efficient if the only deployment objective is to maximise Earth’s overall cooling. However, this would lead to high variance in temperature distributions, namely overcooling of the tropics and undercooling of poles.130 Impacts discussed in Section 3 also can change with a non-equatorial injection — Arctic SAI, for instance, would have less of an effect on Monsoon precipitation.131 Across all these there are key caveats. Neatly framed and optimised objectives found in modelling will not necessarily be reflected in messy and contested real life preferences, nor will SAI necessarily perfectly result in desired “design” outcomes.132
It is also important to consider that SAI may not be used solely to respond to climate change. The multiplicity of potential SAI goals opens the door to hidden agendas,133 self-interest, and misuse. In addition, even if SAI is deployed under an idealistic scenario of climate altruism, there is no guarantee that this would persist. Considering that political preferences are unlikely to remain static over decadal timescales (see Section 5), SAI functions may slowly “creep”134 into currently unknown possibilities of misuse. Such “Function Creep” and potential misuse are highly understudied in current SAI literature.135
Predicting or even foreseeing potential future SAI functions will forever be mired in uncertainty. This is part of why Function Creep is such a difficult policy problem. Initial study in this area for SAI highlights the potential to use SAI to “optimise” or create “designer climates”.136 Actors may, for example, advocate for deployment configurations that lead to more favourable conditions for critical staple crops, especially in response to warming impacts. These decisions may be the product of misjudgement or misinformation on SAI’s causal nature. SAI may also be used to justify the continued existence of fossil fuel industries. This is a potential adverse incentive core to the “moral hazard” problem.137 This could even create a new atmospheric political economy. The fossil fuel industry and other vested interests benefiting from the SAI system would have incentives to both use it as a way to slow decarbonisation and perhaps even thicken SAI deployment over time. This would heighten latent risk. Assuming SAI as a benign climate change response is unwisely narrow. Other more sinister SAI uses, whether purposeful or inadvertent, are critical determinants of SAI’s desirability.
There are many more SAI deployment options that are not currently well captured in extant governance literature. SAI risks for example take a drastically different form if Artificial Intelligence (AI) is one of the central aspects of deployment design. With the vast amounts of information feedback and constant operational adjustments required,138 an advanced deep reinforcement learning system may be used to manage SAI deployment.139 This would introduce a raft of new issues: for instance “black box“ opacity of decision processes140 or inappropriate generalisations of incomplete data.141
Given the high variance of potential SAI objectives and potential deployment configurations, a highly political, uncoordinated, and decentralised142 “Wild West” deployment scenario, with unclear direct impacts, is possible. States and private sector actors are not likely to find agreement on a single defined “set” of objectives, how they should be prioritised, and how these objectives should manifest in deployment configuration. These intensely political and self-interest driven considerations are likely key determinants of SAI deployment impacts, and should be priority areas for future governance research.
The means of deployment for other GCHs also affect SAI risks. An intentional weaponised pandemic may intentionally leverage SAI dynamics, like changes in disease transmission via changes in temperature distribution, to target critical nodes in health and urban systems. Such potential for now is speculative, but ultimately plausible.
7.2 Interconnections
Our analysis has focused on individual pathways for SAI to contribute to GCR. However, none of these are mutually exclusive. Each of the four steams overlap and feed into the same waterway. For instance, uni- or mini-lateral deployment of SRM systems could be driven by geopolitical distrust and conflict. This would likely be a world in which other GCHs are more likely, SAI deployment is less coordinated and damaging, critical systems are less resilient, and the world is less likely to quickly and effectively deal with a termination shock.
There is also an important intersection between systemic and latent risk. Most mechanisms that increase systemic risk will tend to raise latent risk as well. For instance, just in time delivery systems and tightly coupled systems with few back-ups, while efficient, are both more susceptible to shocks and can impede recovery. In Figure 1 we provide one brief attempt to map some of the linkages between different risks and factors in SAI deployment. More interconnected systems mean a higher chance of synchronous failures, and SAI is likely to be a highly interconnected system.
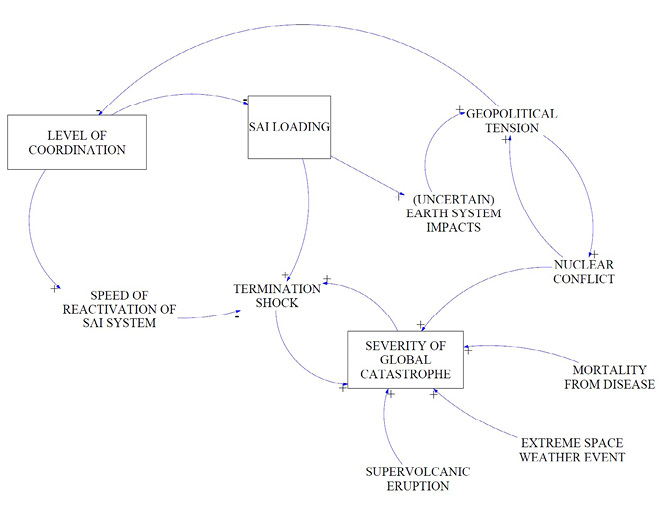
Fig. 1: The SAI-GCR System.143
7.3 Building the Policy Boundaries
Analysis of catastrophic downside risks can help illuminate the contours of what “effective” SAI governance would do. This is a useful complement to the policy literature that has focused mostly on structure and architecture.144 We add to the knowledge on policy instruments by providing further detail on policy approach.
To effectively mitigate against the (limited) number of threats and systemic risks outlined in this chapter, SAI governance would have to be wide ranging, robust, and persist over decades. SAI and its backup infrastructure would need to be built to be resilient to extreme space weather or nuclear EMP events. Effective SAI governance is also not limited to SAI itself, but encompasses other policy areas like health, agriculture, AI, and energy. Ensuring ambitious emissions reductions and greenhouse gas removals would be needed to ensure SAI did not continue indefinitely. Effective SAI governance would also prevent future misuse and balance shifting preferences and multiple deployment goals in a just and inclusive manner. Governance arrangements to ensure SAI deployment or reimplementation in the wake of a major shock like a recession, pandemic, or nuclear attack would also be necessary. These would all be in addition to the herculean technical informational demands necessary for SAI deployment, which alone may be a larger undertaking than an IPCC report.145 This optimistically assumes that SAI’s climatic outputs can be clearly and cleanly measured, that there would be widespread international capacity for effective monitoring,146 and that this monitoring would also be resilient to critical shocks. Substantial advancements in climate science and observation, as well as additional international capacity building for monitoring and transparency, would be needed. All of these would then have to be maintained over the course of decades.
These altogether represent an incredibly challenging governance task. The lack of success of the climate regime, with its similarly intense political and wide-ranging nature, does not inspire confidence in the feasibility of wide-ranging and long-term governance for an issue as political as SAI. Basic discussion over climate engineering as a whole has been stymied under the UN Environment Assembly.147 Future and more consequential SAI debate will be subject to more severe political hurdles. Even less complex and smaller scale governance arrangements, like COVID-19 mask mandates, have been mired in politicisation and competitive dynamics. A “mask” over the Earth, and its associated governance considerations, would face even tougher political challenges to effective implementation.
What would happen if a SAI deployment went ahead without these governance safeguards? It could very well be the case that agricultural or health impacts of SAI are limited or even positive. A disaster like an extreme space weather event may not happen in the decades where SAI is implemented. The stars of international politics could align and allow for a smooth SAI implementation and cessation.
There is indeed no guarantee that the catastrophic pathways outlined in this chapter will materialise. But if they do, they would likely result in severe and cascading consequences. SAI has many extreme downside risks. “Imperfect” SAI governance can be compared to living without health insurance. The extra safeguards and protection aren’t strictly necessary…until something goes wrong. Given what we know about the instability of international geopolitics, SAI with imperfect SAI governance puts the world in a precarious position and introduces a climatic Sword of Damocles. The ultimate question becomes: are we willing to bet the climate that no catastrophe or systemic cascade will trigger SAI’s downside potential over the coming decades?
In a world of imperfect safeguards, two interconnected options are available to alleviate catastrophic risks. The first option is thinner SAI deployment. Thin SAI has a lower risk of catastrophic termination shock, thus posing less of a threat even if triggered by another calamity or systemic cascade. The second option is to ensure diversity in the overall climate engineering portfolio. Reducing reliance on SAI would better allow for a thinner SAI deployment. Other climate engineering approaches, particularly those which are less technology based, would also not necessarily share the same vulnerabilities as SAI. Trees for instance are not vulnerable to extreme space weather. These would reduce the potential of an SAI termination, but ultimately would not completely remedy the political complications SAI would create.
There seem to be three major148 pathways moving forward. The first is living in a highly vulnerable scenario of imperfect SAI governance — the “Damocles Pathway”. This is clearly undesirable. The second is living with well-governed SAI that will not exceed policy boundaries of catastrophe — the “Miracle Pathway”. This seems infeasible. The final middle ground is to accept the inevitably imperfect contours of SAI governance, but greatly limit the extent of SAI deployment — the “Limited Pathway”. But this again would rely on robust and resilient governance and is still vulnerable to geopolitical shocks. SAI may by thickened or thinned along changing political tides.
A core conclusion here is that there is little use in asking whether SAI is a GCR or not. It depends on the level of loading and wider geopolitical landscape. All risks, especially latent risk, will increase with greater loadings and political conflict. This is a critical insight for the wider study of GCRs. A risk cannot be judged in a vacuum. Its severity will inevitably be determined by the scenario and system in which it unfolds.
8. Conclusion: The Frying Pan and the Flame
We map the different contributions of SAI to Global Catastrophic Risk (GCR). The direct risks through irreversible extreme ecosystem impacts are currently unknown. No mechanisms for this have been identified. But extreme ecosystem impacts cannot be confidently ruled out given the nature of the Earth systems. SAI could have numerous diffuse impacts on critical systems such as agriculture, politics and health. These currently appear modest, but we cannot rule out the possibilities of systemic cascades or synchronous failures. It appears unlikely that SAI would trigger any other calamitous hazards unless it ignites geopolitical conflict between great powers. Instead, SAI’s greatest contribution is through latent risk: the ability for termination shock to significantly worsen any other GCR. For each of these areas the evidence base is significantly underdeveloped.
Is SAI worse than the initial problem of climate change? The question for now is largely unanswerable and lies outside the scope of our analysis. This chapter represents a first step in understanding the multitude of risks of SAI. But critical gaps in understanding of both high-end warming and SAI remain. The climate comparison also depends on specific details, such as level of warming, state of politics, and availability of alternatives to SAI (such as rapid large-scale carbon dioxide removal). SAI is also deeply dependent on governance and the level of use. A constrained use of SAI with coherent, coordinated governance would most likely be benign and beneficial. Yet it is in a scenario of extreme warming, political fragmentation, and a search for an escape clause that SAI use appears most likely. Such thick and uncoordinated use of SAI is unwise and an inappropriate precautionary alternative. We would face a planetary Sword of Damocles.
1 The Royal Society. Geoengineering the Climate: Science, Governance and Uncertainty. The Royal Society Publishing (2009). https://doi.org/10.1108/02580541011009815
2 Atkinson, A. Impact Earth: Asteroids, Comets and Meteors — The Growing Threat. Virgin (1999); Global Challenges Foundation, Global Catastrophic Risks Report 2016 (2016).
3 Centeno, Miguel A. et al. ‘The emergence of global systemic risk’, Annual Review of Sociology, 41 (2015): 65–85. https://doi.org/10.1146/annurev-soc-073014-112317
4 Parker, Andy and Peter J. Irvine. ‘The risk of termination shock from solar geoengineering’, Earth’s Future (March 2018): 1–12. https://doi.org/10.1002/2017EF000735
5 Global Challenges Foundation (2016); Bostrom, Nick and Milan M. Ćirković. Global Catastrophic Risks (2008): 554.
6 A single algorithmic system that can perform tasks at a level similar to humans across a broad range of cognitive domains.
7 Avin, Shahar et al. ‘Classifying Global Catastrophic Risks’, Futures (2018). https://doi.org/10.1016/j.futures.2018.02.001; Liu, Hin Yan, Kristian Cedervall Lauta and Matthijs Michiel Maas. ‘Governing boring apocalypses: A new typology of existential vulnerabilities and exposures for existential risk research’, Futures (2018). https://doi.org/10.1016/j.futures.2018.04.009; Baum, Seth D. and Anthony Barrett. ‘Towards an integrated assessment of Global Catastrophic Risk’, Catastrophic and Existential Risk: Proceedings of the First Colloquium (2019): 41–62.
8 IPCC. Managing the Risks of Extreme Events and Disasters to Advance Climate Change Adaptation, ed. Christopher B. Field et al. Cambridge University Press (2012). https://doi.org/10.1017/CBO9781139177245; Currie, Adrian and Seán Ó hÉigeartaigh. ‘Working together to face humanity’s greatest threats: Introduction to the future of research on catastrophic and existential risk’, Futures, 102 (September 2018): 1–5. https://doi.org/10.1016/j.futures.2018.07.003; Liu, Lauta and Maas (2018); Avin et al. (2018).
9 Betz, Gregor. ‘Accounting for possibilities in decision making’, in The Argumentative Turn in Policy Analysis (10th ed.), ed. Sven Ove Hansson and Hirsch Gertrude Hadorn. Springer (2016), pp. 135–69.
10 Wagner, G. and Martin L. Weitzman. Climate Shock: The Economic Consequences of a Hotter Planet. Princeton University Press (2015); Kunreuther, Howard et al. ‘Risk management and climate change’, Nature Climate Change, 3(5) (2013): 447–50. https://doi.org/10.1038/nclimate1740; Ord, Toby, Rafaela Hillerbrand and Anders Sandberg. ‘Probing the improbable: Methodological challenges for risks with low probabilities and high stakes’, Journal of Risk Research, 13(2) (March 2010): 191–205. https://doi.org/10.1080/13669870903126267
11 Homer-Dixon, Thomas et al. ‘Synchronous failure: The emerging causal architecture of global crisis’, Ecology and Society, 20(3) (2015): 1–16.
12 Helbing, Dirk. ‘Globally networked risks and how to respond’, Nature (2013). https://doi.org/10.1038/nature12047; Homer-Dixon, Thomas. The Upside of Down: Catastrophe, Creativity, and the Renewal of Civilization. Island Press (2008).
13 Kemp, Luke et al. ‘Climate endgame: Exploring catastrophic climate change scenarios’, Proceedings of the National Academy of Sciences, 119(34) (23 August 2022). https://doi.org/10.1073/pnas.2108146119
14 McLaren, Duncan. ‘Mitigation deterrence and the “moral hazard” of solar radiation management’, Earth’s Future, 4(12) (2016). https://doi.org/10.1002/2016EF000445
15 McLaren, Duncan P. ‘Whose climate and whose ethics? Conceptions of justice in solar geoengineering modelling’, Energy Research and Social Science (2018). https://doi.org/10.1016/j.erss.2018.05.021
16 Jebari, Joseph et al. ‘From moral hazard to risk-response feedback’, Climate Risk Management, 33 (2021): 100324. https://doi.org/10.1016/j.crm.2021.100324
17 McKinnon, Catriona. ‘Sleepwalking into lock-in? Avoiding wrongs to future people in the governance of solar radiation management research’, Environmental Politics (2018). https://doi.org/10.1080/09644016.2018.1450344
18 This section studies the potential direct catastrophic impact of SAI, not the impacts of termination shock (which are almost guaranteed to be catastrophic if used to mask high levels of warming and if SAI undergoes a indefinite suspension. Trisos, Christopher H. et al. ‘Potentially dangerous consequences for biodiversity of solar geoengineering implementation and termination’, Nature Ecology and Evolution, 2(3) (2018). https://doi.org/10.1038/s41559-017-0431-0. By “directly”, we refer to clear causal relationships with less than two degrees of separation.
19 Reynolds, Jesse L., Andy Parker and Peter Irvine. ‘Five solar geoengineering tropes that have outstayed their welcome Earth’s future’, Earth’s Future, 4 (2016): 562–68. https://doi.org/10.1002/eft2.158; Tilmes, Simone et al. ‘The hydrological impact of geoengineering in the Geoengineering Model Intercomparison Project (GeoMIP)’, Journal of Geophysical Research: Atmospheres, 118(19) (October 2013): 11–36. https://doi.org/10.1002/jgrd.50868
20 Jiang, Jiu et al. ‘Stratospheric sulfate aerosol geoengineering could alter the high-latitude seasonal cycle’, Geophysical Research Letters, 46(23) (December 2019): 14153–63. https://doi.org/10.1029/2019GL085758
21 Dagon, Katherine and Daniel P. Schrag. ‘Exploring the effects of solar radiation management on water cycling in a coupled land–atmosphere model*’, Journal of Climate, 29(7) (April 2016): 2635–50. https://doi.org/10.1175/JCLI-D-15-0472.1; Dagon, Katherine and Daniel P. Schrag. ‘Quantifying the effects of solar geoengineering on vegetation’, Climatic Change (2019). https://doi.org/10.1007/s10584-019-02387-9
22 Zarnetske, Phoebe L. et al. ‘Potential ecological impacts of climate intervention by reflecting sunlight to cool Earth’, Proceedings of the National Academy of Sciences, 118(15) (2021). https://doi.org/10.1073/pnas.1921854118
23 Zarnetske et al. (2021).
24 Trisos et al. (2018); Zarnetske et al. (2021).
25 Lee, Walker Raymond et al. ‘High-latitude stratospheric aerosol geoengineering can be more effective if injection is limited to spring’, Geophysical Research Letters (n.d.). https://doi.org/10.1002/essoar.10505988.1
26 MacMartin, Douglas G. et al. ‘The climate response to stratospheric aerosol geoengineering can be tailored using multiple injection locations’, Journal of Geophysical Research: Atmospheres, 122(23) (December 2017): 12, 512–74, 590. https://doi.org/10.1002/2017JD026868
27 MacMartin, Douglas G. et al. ‘Geoengineering with stratospheric aerosols: what do we not know after a decade of research?’, Earth’s Future (2016). https://doi.org/10.1002/2016EF000418; McCormack, Caitlin G. et al. ‘Key impacts of climate engineering on biodiversity and ecosystems, with priorities for future research’, Journal of Integrative Environmental Sciences (2016). https://doi.org/10.1080/1943815X.2016.1159578; Irvine, Peter J. et al. ‘Towards a comprehensive climate impacts assessment of solar geoengineering’, Earth’s Future, 5(1) (January 2017): 93–106. https://doi.org/10.1002/2016EF000389; Irvine, Peter J. et al. ‘An overview of the Earth system science of solar geoengineering’, Wiley Interdisciplinary Reviews: Climate Change (2016). https://doi.org/10.1002/wcc.423; Schäfer, Stefan et al. The European Transdisciplinary Assessment of Climate Engineering (2015).
28 Brysse, Keynyn et al. ‘Climate change prediction: Erring on the side of least drama?’, Global Environmental Change, 23(1) (2013): 327–37. https://doi.org/10.1016/j.gloenvcha.2012.10.008
29 Jehn, Florian Ulrich et al. ‘Betting on the best case: Higher end warming is underrepresented in research’, Environmental Research Letters, 16(8) (1 August 2021): 084036. https://doi.org/10.1088/1748-9326/ac13ef; Geden, Oliver. ‘Policy: Climate advisers must maintain integrity’, Nature, 521(7550) (May 2015): 27–28. https://doi.org/10.1038/521027a
30 Low, Sean and Matthias Honegger. ‘A precautionary assessment of systemic projections and promises from sunlight reflection and carbon removal modeling’, Risk Analysis (n.d.). https://doi.org/10.1111/risa.13565
31 Irvine, Peter et al. ‘Halving warming with idealized solar geoengineering moderates key climate hazards’, Nature Climate Change (March 2019). https://doi.org/10.1038/s41558-019-0398-8
32 Lee et al. (n.d.).
33 Abatayo, Anna Lou et al. ‘Solar geoengineering may lead to excessive cooling and high strategic uncertainty’, Proceedings of the National Academy of Sciences, 117(24) (2020): 13393–98. https://doi.org/10.1073/pnas.1916637117
34 Kravitz, Ben et al. ‘Sulfuric acid deposition from stratospheric geoengineering with sulfate aerosols’, Journal of Geophysical Research: Atmospheres, 114(D14) (July 2009). https://doi.org/10.1029/2009JD011918; Visioni, Daniele et al. ‘What goes up must come down: Impacts of deposition in a sulfate geoengineering scenario’, Environmental Research Letters (2020). https://doi.org/10.1088/1748-9326/ab94eb
35 Commonly proposed coolant agents could be benign or even increase ozone thickness. Irvine et al. (2016); Pitari, Giovanni et al. ‘Stratospheric ozone response to sulfate geoengineering: results from the Geoengineering Model Intercomparison Project (GeoMIP)’, Journal of Geophysical Research: Atmospheres, 119(5) (March 2014): 2629–53. https://doi.org/10.1002/2013JD020566 (though this is a relatively recent shift).
36 Heckendorn, P. et al. ‘The impact of geoengineering aerosols on stratospheric temperature and ozone’, Environmental Research Letters (2009). https://doi.org/10.1088/1748-9326/4/4/045108; Keith, David W. et al. ‘Stratospheric solar geoengineering without ozone loss’, Proceedings of the National Academy of Sciences, 113(52) (2016): 14910–14. https://doi.org/10.1073/pnas.1615572113
37 Halstead, John. ‘Stratospheric aerosol injection research and existential risk’, Futures, 102 (March 2018): 63–77. https://doi.org/10.1016/j.futures.2018.03.004
38 Elsawah, Sondoss et al. ‘Eight grand challenges in socio-environmental systems modeling’, Socio-Environmental Systems Modelling, 2 (January 2020): 16226. https://doi.org/10.18174/sesmo.2020a16226
39 A “free-driver” problem (or “forced rider”) is where a single or limited number of actors’ actions create negative consequences that the rest of the system must endure. In this case, a limited number of actors could implement SAI, and the rest of the world’s economic and political systems would have to deal with the consequences. This can be seen as the “opposite“ to a conventional “free rider” problem where benefits flow onto non-cooperative third parties,
40 Weitzman, Martin L. ‘A voting architecture for the governance of free-driver externalities, with application to geoengineering’, Scandinavian Journal of Economics, 117(4) (2015): 1049–68. https://doi.org/10.1111/sjoe.12120
41 Seto, Karen C. et al. ‘Carbon lock-in: Types, causes, and policy implications’, Annual Review of Environment and Resources, 41(1) (October 2016): 425–52. https://doi.org/10.1146/annurev-environ-110615-085934
42 Williams, John W., Alejandro Ordonez and Jens-Christian Svenning. ‘A unifying framework for studying and managing climate-driven rates of ecological change’, Nature Ecology & Evolution, 5(1) (2021): 17–26. https://doi.org/10.1038/s41559-020-01344-5; Williams, John W. and Stephen T. Jackson. ‘Novel climates, no-analog communities, and ecological surprises’, Frontiers in Ecology and the Environment, 5(9) (November 2007): 475–82. https://doi.org/10.1890/070037
43 McKinnon (2018).
44 Baum, Seth, Timothy M. Maher Jr. and Jacob Haqq-Misra. ‘Double catastrophe: Intermittent stratospheric geoengineering induced by societal collapse’, Environment, Systems and Decisions, 33(1) (2013): 1–21.
45 Instead of Global Catastrophic Risks, this section focuses on Global Catastrophic Hazards. Risks include vulnerability and exposure. Instead, this section focuses on the interactions between different specific hazards and SAI.
46 Phillips, Carly A. et al. ‘Compound climate risks in the COVID-19 pandemic’, Nature Climate Change, 10(7) (2020): 586–88. https://doi.org/10.1038/s41558-020-0804-2
47 Baum, Maher Jr. and Haqq-Misra (2013).
48 Laakso, A. et al. ‘Radiative and climate impacts of a large volcanic eruption during stratospheric sulfur geoengineering’, Atmospheric Chemistry and Physics, 16(1) (January 2016): 305–23. https://doi.org/10.5194/acp-16-305-2016
49 MacMartin, Douglas G. et al. ‘Technical characteristics of a solar geoengineering deployment and implications for governance’, Climate Policy, 19(10) (November 2019): 1325–39. https://doi.org/10.1080/14693062.2019.1668347
50 MacMartin et al. (2019).
51 MacMartin et al. (2019).
52 Mani, Lara, Asaf Tzachor and Paul Cole. ‘Global Catastrophic Risk from lower magnitude volcanic eruptions’, Nature Communications, 12(1) (6 December 2021): 4756. https://doi.org/10.1038/s41467-021-25021-8
53 Green, James L. and Scott Boardsen. ‘Duration and extent of the great auroral storm of 1859’, Advances in Space Research, 38(2) (2006): 130–35. https://doi.org/10.1016/j.asr.2005.08.054
54 Eastwood, J. P. et al. ‘The economic impact of space weather: where do we stand?’, Risk Analysis, 37(2) (February 2017): 206–18. https://doi.org/10.1111/risa.12765; Ritter, Scott et al. ‘International legal and ethical issues of a future carrington event: Existing frameworks, shortcomings, and recommendations’, New Space, 8(1) (2020): 23–30. https://doi.org/10.1089/space.2019.0026; Loper, Robert D. ‘Carrington-class events as a great filter for electronic civilizations in the drake equation’, Publications of the Astronomical Society of the Pacific, 131(998) (April 2019): 044202. https://doi.org/10.1088/1538-3873/ab028e
55 There is unresolved discussion as to whether extreme space weather events follow a power law or lognormal distribution. Kataoka, Ryuho. ‘Extreme geomagnetic activities: A statistical study’, Earth, Planets and Space, 72(1) (2020): 124. https://doi.org/10.1186/s40623-020-01261-8; Riley, Pete and Jeffrey J. Love. ‘Extreme geomagnetic storms: Probabilistic forecasts and their uncertainties’, Space Weather, 15(1) (January 2017): 53–64. https://doi.org/10.1002/2016SW001470. The estimated probabilities of an extreme space weather event in the next decade or so range from 0.46 % to 1.88 %. Moriña, David et al. ‘Probability estimation of a Carrington-like geomagnetic storm’, Scientific Reports, 9(1) (2019): 2393. https://doi.org/10.1038/s41598-019-38918-8; 4–6 %, Kataoka, Ryuho. ‘Probability of occurrence of extreme magnetic storms’, Space Weather, 11(5) (May 2013): 214–18. https://doi.org/10.1002/swe.20044, to roughly 20.3 %. Riley and Love (2017). This is all to say that while there are efforts to better understand the probability of extreme space weather events, there is little agreement in what the precise probability is. Nonetheless, even the lowest estimates are not negligible. In the face of such uncertainty, we take the lead of other policy analyses in the area. Royal Academy of Engineering. Extreme Space Weather: Impacts on Engineered Systems and Infrastructure (2013); National Science and Technology Council. National Space Weather Strategy and Action Plan (2019) and characterise these events as essentially random.
56 Royal Academy of Engineering (2019).
57 Jones, J. B. L. et al. ‘Space weather and commercial airlines’, Advances in Space Research, 36(12) (2005): 2258–67. https://doi.org/10.1016/j.asr.2004.04.017
58 Goodman, John M. ‘Operational communication systems and relationships to the ionosphere and space weather’, Advances in Space Research, 36(12) (2005): 2241–52. https://doi.org/10.1016/j.asr.2003.05.063; Royal Academy of Engineering (2019).
59 Jones et al. (2005).
60 Alvarez, Luis E., Sebastian D. Eastham and Steven R. H. Barrett. ‘Radiation dose to the global flying population’, Journal of Radiological Protection, 36(1) (March 2016): 93–103. https://doi.org/10.1088/0952-4746/36/1/93; Dyer, C. S. et al. ‘Solar particle enhancements of single-event effect rates at aircraft altitudes’, IEEE Transactions on Nuclear Science, 50(6) (December 2003): 2038–45. https://doi.org/10.1109/TNS.2003.821375; Jones et al. (2005).
61 Jones et al. (2005).
62 Royal Academy of Engineering (2019); National Science and Technology Council (2019).
63 Liu, Ying D. et al. ‘Observations of an extreme storm in interplanetary space caused by successive coronal mass ejections’, Nature Communications, 5(1) (2014): 3481. https://doi.org/10.1038/ncomms4481; Pulkkinen, Antti et al. ‘Regional-scale high-latitude extreme geoelectric fields pertaining to geomagnetically induced currents’, Earth, Planets and Space, 67(1) (2015): 93. https://doi.org/10.1186/s40623-015-0255-6; Eastwood et al. (2017).
64 Eroshenko, E. A. et al. ‘Effects of strong geomagnetic storms on northern railways in Russia’, Advances in Space Research, 46(9) (2010): 1102–10. https://doi.org/10.1016/j.asr.2010.05.017; Wik, M. et al. ‘Space weather events in july 1982 and october 2003 and the effects of geomagnetically induced currents on Swedish technical systems’, Annales Geophysicae, 27(4) (2009): 1775–87. https://doi.org/10.5194/angeo-27-1775-2009; Ptitsyna, N. G. et al. ‘Geomagnetic effects on mid-latitude railways: A statistical study of anomalies in the operation of signaling and train control equipment on the East-Siberian Railway’, Advances in Space Research, 42(9) (2008): 1510–14. https://doi.org/10.1016/j.asr.2007.10.015
65 Juusola, L. et al. ‘High-latitude ionospheric equivalent currents during strong space storms: Regional perspective’, Space Weather — The International Journal Of Research And Applications, 13(1) (January 2015): 49–60. https://doi.org/10.1002/2014SW001139; Wang, Kai-rang, L. I. U. Lian-guang and L. I. Yan. ‘Preliminary analysis on the interplanetary cause of geomagnetically induced current and its effect on power systems’, Chinese Astronomy and Astrophysics, 39(1) (2015): 78–88. https://doi.org/10.1016/j.chinastron.2015.01.003; Matandirotya, Electdom, Pierre J. Cilliers and Robert R. Van Zyl. ‘Modeling geomagnetically induced currents in the South African power transmission network using the finite element method’, Space Weather, 13(3) (March 2015): 185–95. https://doi.org/10.1002/2014SW001135; Royal Academy of Engineering (2019).
66 Odenwald, Sten, James Green and William Taylor. ‘Forecasting the impact of an 1859-calibre superstorm on satellite resources’, Advances in Space Research, 38(2) (2006): 280–97. https://doi.org/10.1016/j.asr.2005.10.046
67 Loper (2019).
68 Loper (2019).
69 MacMartin et al. (2019).
70 National Science and Technology Council (2019).
71 Barrett, Anthony M., Seth D. Baum and Kelly Hostetler. ‘Analyzing and reducing the risks of inadvertent nuclear war between the United States and Russia’, Science & Global Security, 21(2) (May 2013): 106–33. https://doi.org/10.1080/08929882.2013.798984; Baum, Seth, Robert de Neufville and Anthony Barrett. ‘A model for the probability of nuclear war’, SSRN Electronic Journal (2018). https://doi.org/10.2139/ssrn.3137081
72 Baum, de Neufville and Barrett (2018).
73 Baum, Maher Jr. and Haqq-Misra (2013).
74 Edwards, Paul N. ‘Entangled histories: Climate science and nuclear weapons research’, Bulletin of the Atomic Scientists, 68(4) (July 2012): 28–40. https://doi.org/10.1177/0096340212451574
75 Jinnah, Sikina. ‘Building a governance foundation for solar geoengineering deployment’, in Governance of the Deployment of Solar Geoengineering, ed. Robert N. Stavins and Robert Stowe. Harvard Project on Climate Agreements (2019), pp. 143–48; Horton, J. B. and J. L. Reynolds. ‘The international politics of climate engineering: a review and prospectus for international relations’, International Studies Review, 18(3) (2016): 438–61. https://doi.org/10.1093/isr/viv013; Chhetri, Netra et al. Governing Solar Radiation Management (2018). https://doi.org/10.17606/M6SM17
76 McLaren, Duncan and Olaf Corry. ‘Clash of geofutures and the remaking of planetary order: Faultlines underlying conflicts over geoengineering governance’, Global Policy, 12(S1) (April 2021): 20–33. https://doi.org/10.1111/1758-5899.12863
77 Doan, Xuan Vinh and Duncan Shaw. ‘Resource allocation when planning for simultaneous disasters’, European Journal of Operational Research, 274(2) (2019): 687–709. https://doi.org/10.1016/j.ejor.2018.10.015; Platt, Rutherford H. Disasters and Democracy: The Politics of Extreme Natural Events. Island Press (1999); Cohen, Charles and Eric D. Werker. ‘The political economy of “natural’’ disasters’, Journal of Conflict Resolution, 52(6) (December 2008): 795–819. https://doi.org/10.1177/0022002708322157
78 Baum, Maher Jr. and Haqq-Misra (2013).
79 Carlson, Colin J. et al. ‘Solar geoengineering could redistribute malaria risk in developing countries’, MedRxiv (2020). https://doi.org/10.1101/2020.10.21.20217257; Carlson, Colin J. and Christopher H. Trisos. ‘Climate engineering needs a clean bill of health’, Nature Climate Change, 8(10) (2018): 843–45. https://doi.org/10.1038/s41558-018-0294-7
80 Christophersen, Olav Albert and Anna Haug. ‘Why is the world so poorly prepared for a pandemic of hypervirulent avian influenza?’, Microbial Ecology in Health and Disease, 18(3–4) (January 2006): 113–32. https://doi.org/10.1080/08910600600866544; Morens, David M. et al. ‘The origin of COVID-19 and why it matters’, The American Journal of Tropical Medicine and Hygiene, 103(3) (September 2020): 955–59. https://doi.org/10.4269/ajtmh.20-0849; Cheng, Vincent C. C. et al. ‘Severe acute respiratory syndrome coronavirus as an agent of emerging and reemerging infection’, Clinical Microbiology Reviews (2007). https://doi.org/10.1128/CMR.00023-07; Quammen, David. Spillover: Animal Infections and the Next Human Pandemic. W. W. Norton & Company (2012); Plowright, Raina K. et al. ‘Pathways to Zoonotic spillover’, Nature Reviews Microbiology, 15(8) (2017): 502–10. https://doi.org/10.1038/nrmicro.2017.45; Johnson, Christine K. et al. ‘Global shifts in mammalian population trends reveal key predictors of virus spillover risk’, Proceedings of the Royal Society B: Biological Sciences, 287(1924) (April 2020): 20192736. https://doi.org/10.1098/rspb.2019.2736; Borremans, Benny et al. ‘Cross-species pathogen spillover across ecosystem boundaries: Mechanisms and theory’, Philosophical Transactions of the Royal Society B: Biological Sciences, 374(1782) (September 2019): 20180344. https://doi.org/10.1098/rstb.2018.0344
81 Helbing (2013); Homer-Dixon (2008); Haldane, Andrew G. and Robert M. May. ‘Systemic risk in banking ecosystems’, Nature, 469(7330) (2011): 351–55. https://doi.org/10.1038/nature09659
82 Helbing (2013).
83 Russon, Mary-Ann. ‘The cost of the Suez Canal blockage’, BBC News (2021).
84 We exclude other areas such as telecommunications and finance due to a lack of published literature.
85 Kortetmäki, Teea and Markku Oksanen. ‘Food systems and climate engineering : A plate full of risks or promises?’, Climate Justice and Geoengineering. Rowman & Littlefield International (2016), pp. 121–35; Pamplany, Augustine, Bert Gordijn and Patrick Brereton. ‘The ethics of geoengineering: A literature review’, Science and Engineering Ethics, 26(6) (2020): 3069–3119. https://doi.org/10.1007/s11948-020-00258-6; Svoboda, Toby et al. ‘The potential for climate engineering with stratospheric sulfate aerosol injections to reduce climate injustice’, Journal of Global Ethics, 14(3) (2018). https://doi.org/10.1080/17449626.2018.1552180; Irvine et al. (2017).
86 Xia, Lilia et al. ‘Solar radiation management impacts on agriculture in China: A case study in the Geoengineering Model Intercomparison Project (GeoMIP)’, Journal of Geophysical Research: Atmospheres, 119(14) (July 2014): 8695–8711. https://doi.org/10.1002/2013JD020630
87 Pongratz, J. et al. ‘Crop yields in a geoengineered climate’, Nature Climate Change, 2(2) (2012): 101–5. https://doi.org/10.1038/nclimate1373
88 Yang, Huiyi et al. ‘Potential negative consequences of geoengineering on crop production: A study of Indian groundnut’, Geophysical Research Letters, 43(22) (November 2016): 11,711–86,795. https://doi.org/10.1002/2016GL071209
89 Proctor, Jonathan et al. ‘Estimating global agricultural effects of geoengineering using volcanic eruptions’, Nature, 560(7719) (2018): 480–83. https://doi.org/10.1038/s41586-018-0417-3
90 Richards, C. E., R. C. Lupton and J. M. Allwood. ‘Re-framing the threat of global warming: An empirical causal loop diagram of climate change, food insecurity and societal collapse’, Climatic Change, 164(3) (2021): 49. https://doi.org/10.1007/s10584-021-02957-w; Natalini, Davide, Giangiacomo Bravo, and Aled Wynne Jones. ‘Global food security and food riots — An agent-based modelling approach’, Food Security, 11 (2017): 1153–73. https://doi.org/10.1007/s12571-017-0693-z; Natalini, Davide, Aled Wynne Jones and Giangiacomo Bravo. ‘Quantitative assessment of political fragility indices and food prices as indicators of food riots in countries’, Sustainability, 7(4) (2015): 4360–85. https://doi.org/10.3390/su7044360
91 Kortetmäki and Oksanen (2016).
92 Proctor et al. (2018).
93 Xu, Chi et al. ‘Future of the human climate niche’, Proceedings of the National Academy of Sciences (2020): 1–6. https://doi.org/10.1073/pnas.1910114117; Burke, Marshall, Solomon M. Hsiang and Edward Miguel. ‘Global non-linear effect of temperature on economic production’, Nature, 527(7577) (2015): 235–39. https://doi.org/10.1038/nature15725
94 McKinnon, Catriona. ‘The Panglossian politics of the geoclique’, Critical Review of International Social and Political Philosophy, 23(5) (2020): 584–99. https://doi.org/10.1080/13698230.2020.1694216; Horton and Reynolds (2016).
95 Corry, Olaf. ‘The international politics of geoengineering: The feasibility of Plan B for tackling climate change’, Security Dialogue, 48(4) (2017): 297–315. https://doi.org/10.1177/0967010617704142; Keith, David. A Case for Climate Engineering. MIT Press (2013).
96 MacMartin et al. (2019).
97 Horton and Reynolds (2016); Fleming, James Rodger. ‘The climate engineers’, The Wilson Quarterly, 31(2) (2007): 46–60. https://doi.org/10.2307/40262106; Lin, Albert C. ‘The missing pieces of geoengineering research governance’, Minnesota Law Review, 100 (2016): 2509–76; Olson, R. ‘Geoengineering for decision makers’, Science and Technology Innovation Program (2011); Halstead (2018).
98 BBC, ‘US fuel pipeline “paid hackers $5m in ransom”’, BBC News (2021).
99 Rolnick, David et al. ‘Tackling climate change with machine learning’, ArXiv (2019); de Witt, Christian Schroeder and Thomas Hornigold. ‘Stratospheric aerosol injection as a deep reinforcement learning problem’, ArXiv (2019).
100 MacMartin et al. (2019).
101 Slay, Jill and Michael Miller. Lessons Learned from the Maroochy Water Breach BT — Critical Infrastructure Protection, ed. Eric Goetz and Sujeet Shenoi. Springer US (2008), pp. 73–82.
102 Wells, Linton. Thoughts for the 2001 Quadrennial Defense Review (2001).
103 Carlson and Trisos (2018).
104 Carlson et al. (2020); Carlson and Trisos (2018).
105 Effiong, Utibe and Richard L. Neitzel. ‘Assessing the direct occupational and public health impacts of solar radiation management with stratospheric aerosols’, Environmental Health, 15(1) (December 2016): 7. https://doi.org/10.1186/s12940-016-0089-0; Eastham, Sebastian D. et al. ‘Quantifying the impact of sulfate geoengineering on mortality from air quality and UV-B exposure’, Atmospheric Environment, 187 (2018): 424–34. https://doi.org/10.1016/j.atmosenv.2018.05.047; Carlson et al. (2020); Carlson and Trisos (2018).
106 Effiong and Neitzel (2016).
107 Eastham et al. (2018).
108 Carlson and Trisos (2018).
109 Cotton-Barratt, Owen, Max Daniel and Anders Sandberg. ‘Defence in depth against human extinction: Prevention, response, resilience, and why they all matter’, Global Policy, 11(3) (1 May 2020): 271–82. https://doi.org/10.1111/1758-5899.12786
110 Kosugi, Takanobu. ‘Fail-safe solar radiation management geoengineering’, Mitigation and Adaptation Strategies for Global Change, 18(8) (2013): 1141–66. https://doi.org/10.1007/s11027-012-9414-2
111 Parker and Irvine (2018).
112 Lynas, Mark. Our Final Warning: Six Degrees of Climate Emergency. Harper Collins (2020).
113 Parker and Irvine (2018); Reynolds, Parker and Irvine (2016).
114 Ott, Konrad K. ‘On the political economy of solar radiation management strategies to combat impacts of climate’, Frontiers in Environmental Science, 6 (June 2018): 1–13. https://doi.org/10.3389/fenvs.2018.00043
115 Moriña et al. (2019).
116 Riley and Love (2017).
117 Barrett, Baum and Hostetler (2013).
118 Reynolds, Parker and Irvine (2016).
119 Parker and Irvine (2018).
120 Castillo, Juan Camilo et al. ‘Market design to accelerate COVID-19 vaccine supply’, Science, 371(6534) (12 March 2021): 1107–9. https://doi.org/10.1126/science.abg0889
121 Homer-Dixon et al. (2015).
122 Parker and Irvine (2018); McKinnon (2020); Halstead (2018).
123 Rabitz, Florian. ‘Governing the termination problem in solar radiation management’, Environmental Politics, 28(3) (2019): 502–22. https://doi.org/10.1080/09644016.2018.1519879; Parker and Irvine (2018).
124 Maas, Matthijs M. ‘How viable is international arms control for military artificial intelligence? Three lessons from nuclear weapons’, Contemporary Security Policy, 40(3) (July 2019): 285–311. https://doi.org/10.1080/13523260.2019.1576464; Perrow, Charles. Normal Accidents: Living With High Risk Technologies — Updated Edition. Princeton University Press (1999).
125 Lee, W. et al. ‘Expanding the design space of stratospheric aerosol geoengineering to include precipitation-based objectives and explore trade-offs’, Earth System Dynamics, 11(4) (2020): 1051–72. https://doi.org/10.5194/esd-11-1051-2020; Zarnetske et al. (2021).
126 Kravitz, Ben et al. ‘Geoengineering as a design problem’, Earth System Dynamics, 7(2) (May 2016): 469–97. https://doi.org/10.5194/esd-7-469-2016
127 MacMartin et al. (2019); Kravitz, Ben et al. ‘Comparing surface and stratospheric impacts of geoengineering with different SO2 injection strategies’, Journal of Geophysical Research: Atmospheres, 124(14) (July 2019): 7900–18. https://doi.org/10.1029/2019JD030329; MacMartin et al. (2017); Keith et al. (2016); Pope, F. D. et al. ‘Stratospheric aerosol particles and solar-radiation management’, Nature Climate Change, 2(10) (2012): 713–19. https://doi.org/10.1038/nclimate1528; Visioni, Daniele et al. ‘Seasonally modulated stratospheric aerosol geoengineering alters the climate outcomes’, Geophysical Research Letters, 47(12) (June 2020): e2020GL088337. https://doi.org/10.1029/2020GL088337
128 Bala, G. ‘Problems with geoengineering schemes to combat climate change’, Current Science, 96(1) (2009): 41–48. https://doi.org/10.1006/asle.2002.0099; Krishnamohan, K. S. et al. ‘The climatic effects of hygroscopic growth of sulfate aerosols in the stratosphere’, Earth’s Future, 8(2) (February 2020): e2019EF001326. https://doi.org/10.1029/2019EF001326
129 MacMartin et al. (2017).
130 MacMartin et al. (2017).
131 Sun, Weiyi et al. ‘Global monsoon response to tropical and arctic stratospheric aerosol injection’, Climate Dynamics, 55(7) (2020): 2107–21. https://doi.org/10.1007/s00382-020-05371-7
132 Wiertz, Thilo. ‘Visions of climate control’, Science, Technology, & Human Values, 41(3) (May 2016): 438–60. https://doi.org/10.1177/0162243915606524; McLaren (2018).
133 McConnell, Allan. ‘Hidden agendas: Shining a light on the dark side of public policy’, Journal of European Public Policy, 25(12) (December 2018): 1739–58. https://doi.org/10.1080/13501763.2017.1382555
134 Koops, Bert-Jaap. ‘The concept of function creep’, Law, Innovation and Technology, 13(1) (January 2021): 29–56. https://doi.org/10.1080/17579961.2021.1898299
135 There is the ethical question of how research in SAI misuse should be undertaken. Will researching SAI misuse possibilities inadvertently encourage SAI misuse? This is a question beyond the scope of this chapter but is regardless a critical issue that deserves future attention.
136 Talberg, Anita, Sebastian Thomas and John Wiseman. ‘A scenario process to inform australian geoengineering policy’, Futures (June 2018). https://doi.org/10.1016/j.futures.2018.06.003; Preston, Christopher J. ‘Ethics and geoengineering: Reviewing the moral issues raised by solar radiation management and carbon dioxide removal’, Wiley Interdisciplinary Reviews: Climate Change, 4(1) (2013): 23–37. https://doi.org/10.1002/wcc.198
137 Lockley, Andrew and D’Maris Coffman. ‘Distinguishing morale hazard from moral hazard in geoengineering’, Environmental Law Review (2016). https://doi.org/10.1177/1461452916659830; Reynolds, Jesse. ‘A critical examination of the climate engineering moral hazard and risk compensation concern’, Anthropocene Review, 2(2) (2015): 174–91. https://doi.org/10.1177/2053019614554304
138 MacMartin et al. (2019).
139 Rolnick et al. (2019); Schroeder de Witt and Hornigold (2019).
140 Rudin, Cynthia and Joanna Radin. ‘Why are we using black box models in ai when we don’t need to? a lesson from an explainable ai competition’, Harvard Data Science Review, 1(2) (November 2019). https://doi.org/10.1162/99608f92.5a8a3a3d
141 Christie, Alec P. et al. ‘The challenge of biased evidence in conservation’, Conservation Biology (2020). https://doi.org/10.1111/cobi.13577; Martin, Laura J., Bernd Blossey and Erle Ellis. ‘Mapping where ecologists work: Biases in the global distribution of terrestrial ecological observations’, Frontiers in Ecology and the Environment, 10(4) (2012): 195–201. https://doi.org/10.1890/110154
142 Reynolds, Jesse L. and Gernot Wagner. ‘Highly decentralized solar geoengineering’, Environmental Politics (July 2019): 1–17. https://doi.org/10.1080/09644016.2019.1648169
143 This is a causal loop diagram. A complete line represents a positive polarity (meaning an amplifying feedback, not necessarily positive in a normative sense) and a dotted line denotes a negative polarity (meaning a dampening feedback).
144 Reynolds, Jesse L. ‘Solar geoengineering to reduce climate change: A review of governance proposals’, Proceedings of the Royal Society A: Mathematical, Physical and Engineering Sciences (2019). https://doi.org/10.1098/rspa.2019.0255
145 MacMartin et al. (2019).
146 McLaren (2018).
147 McLaren and Corry (2021).
148 There are likely going to be many ”in-betweens”, but these seem like the major contours of SAI’s future.